
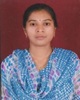
- Open Access
- Authors : Shraddha Mahakal , Sachin Suse
- Paper ID : IJERTV9IS120028
- Volume & Issue : Volume 09, Issue 12 (December 2020)
- Published (First Online): 14-12-2020
- ISSN (Online) : 2278-0181
- Publisher Name : IJERT
- License:
This work is licensed under a Creative Commons Attribution 4.0 International License
Amino acid Adapted Metal and Metals Oxides
Shraddha Mahakal1
1 Department of Physics, Annasaheb Awate College, Manchar,
Pune. 410503(MS) India.
Sachin Suse2
2 Department of B. Voc.
(Food Processing & Quality Management) Annasaheb Awate College, Manchar, Pune.410503(MS) India.
Abstract The smart and multifunctional use of emerging material having novel properties in a green way is the demands of todays life. The quest of new material has fueled the material science in a great extent. The selection of proper material, synthesis, analysis, modification in properties and application are critical on the platform of environment friendly. Traditionally inorganic materials were adopted in connection with synthesis, analysis and application. But intervening of organic material or biomolecules in the inorganic material is playing the pivot role in properties and hence has scope in the application context.
Biomolecules like amino acids are available on earth in different forms. Amino Acids are the integral part of life on earth as they are significant often called as building blocks of proteins. They can use for structure directing agents or tailoring the properties for the suitable modification of morphology in the specific application purpose. This bio-composite is eco-friendly too. So here in the present review paper we have shed a light on the synthesis methods at different pH, temperature, binding of amino acid to the host and application on metal and semiconductor oxide and calcite modified with various amino acids.
Keywords Amino acids, Metals, Oxidation, Bioimolecules, Nanoparticles, Biomineralization.
INTRODUCTION
(Metal and metal oxide Nanoparticles)
Metal and metal oxide Nanoparticles (NPs) have achieved significant position in the various fields of sciences including, physics, chemistry, pharmaceutical and biology etc. The advancement in research of material science has made available the new and versatile materials with suitable activity and properties. We can tune size of the particles which alters the properties of the nanoparticles. These nanoparticles have successfully revolutionized in the sector of energy sector too [1]. Gold nanoparticles are used in drug delivery in cancer cells [2] and bio-sensing [3]. The modification and modulation of crystal properties by proteins are common phenomena in living organisms. The regulation of hard tissues in teeth, bones mollusk shells are natural examples of such type.
The behavior of inorganic nanomaterials in response to biomolecules is tedious because of complex nature of biomolecule. Therefore it is necessary to consider a simple representative system for this interaction. So we have selected only metal, semiconductor and calcite as inorganic material and amino acid as a biomolecule. Metal [4,5] and semiconductor nanoparticles [6] are showing excellent applications in electronic devices, photocatalyst, sensors, antibacterial and diagnostic applications. TiO2 nanoparticles are major constituents in sunscreen lotion as it absorbs UV light [7]. ZnO nanoparticles have wide applications in
piezoelectric [8] and optoelectronic [9] applications. Metal nanoparticles like gold and silver, semiconductor nanoparticles like ZnO, TiO2, CuO and minerals like Calcite (Calcium Carbonate) has unique applications in this context.
In the recent era of technology these metal and semiconductor nanoparticles are building blocks of small electronic components. Therefore the use of nanomaterials in most of the field has created new topic of research about influence of nanomaterials on environment ecosystem. Scientists are indulged to make these nanoparticles biocompatible and functional by use of biomolcules like polymers or amino acid or surfactants like CTAB and PVP etc. The uses of biomolecules or surfactants are responsible for the variation of shape and size of nanomaterials in this regard [10, 11]. The use of polymer also acts as surfactant of the ZnO host material [12, 13]. The interaction of these inorganic nanoparticles with the biomolecules is governed by pH, charge, temperature, size, shape and type of biomolcules at the interface.
Maintaining the nano-size and also the colloidal stability is extremely challenging. The major challenge can be tackled through the use of suitable capping or doping agents. Some of the amino acids (AA) namely histidine, serine, cysteine etc., are suitable chelators for metal based nano-particles like zinc
[14] and cadmium [15]. Amino acids are having tendency to modify the morphology of nanoparticles [16]. Since amino acids are naturally occurring biomolecule with abundance in the root zone of the plat (rhizosphere). They are comprising of1) Amino group (-NH2) 2) Carboxyl group (COOH) and 3) Side chain (-R) group. Thus, amino acids have three functional groups which can react with inorganic nanoparticles. In this manner interfacings of organic and inorganic compounds are having cardinal importance in order to understand biosensors, biomedical implants or other organometallic compounds. The inter-linkage of organic with inorganic compound has found in nature like in zinc finger in which amino acid is coupled with ZnO. The representative assemble of organic and inorganic compound in living organism is biomineralization in which metal ion is linked with biomolecule [17].
Amino acids are building blocks of proteins, enzymes and muscle tissue which are required to all animals for proper functioning of body and allied physiological processes. The simplicity of interaction of biomolecules with inorganic material has benefits: 1) Amino acids can influence the precise control on shape, size, crystallinity or other chemical property of inorganic materials 2) This reaction takes place at low temperature with organic solvent 3) Diverse types of amino acid are available 4) Modification of morphology and capping enable to alter physical property of inorganic material [18].
On an account of above discussion, we have considered metal binding properties of amino acid for some metals nanoparticles like Au, Ag and semiconductor nanoparticles like TiO2, ZnO and Calcite. There are 20 natural amino acids so diverse metal binding alternatives are available. There are several amino acids which are used as reducing and capping agents in the metal nanoparticles synthesis [19, 20, 21].
GOLD AND SILVER NANOPARTICLES:
Gold and silver nanoparticles are tremendous application in the biomedical field like bio-imaging [22], targeted drug delivery [23] and bio-sensing [24]. The use of gold nanoparticles in biomedical field is owing to the unique Surface Plasmon Resonance (SPR) property of gold nanoparticles which can be tuned by size of nanoparticles. The activity of proteins in the formation gold nanoparticles has studied [25].
AMINO ACID INTERFACED GOLD NANOPARTICLES:
From Roman times gold nanoparticles were used to stain glasses with beautiful shades of colors. Faraday in 1857 reported colloidal gold nanoparticles [4]. By considering the implementation of gold nanoparticles in biomedical field which needs benign functionalization instead of harsh chemicals and biocompatible. Amino acid can be used as a reducing and capping agent for metals, semiconductors and insulator [26, 27]. Simulation study of binding preferences on gold nanoparticles were analyzed [28,29]. The unique linking between nanoparticles with amino acid is governed by pH of the solution. At acidic (low) pH Au nanoparticles linked by amine group (near to carboxylic acid group COOH) while at high pH due to repulsion between Au surfaces and negatively charged -caboxylic group this bonding is not takes place [30]. The detailed investigation between gold nanoparticles with amine group of amino acid has been studied. They proposed the interaction of amino acid and Au nanoparticles via amine group and Cross-Linking of Au Colloids via Hydrogen Bonding in Thiols Containing OH Groups [31]. Amine stabilized gold nanoparticles were also analyzed [32]. Tryptophan has been act as a reducing agent [33]. The inherent property of gold nanoparticles to bind and form complex with amino acid can be used to detect amino acid if proper functionalized. Quercetin-functionalized gold nanoparticles are capable of visual detection of amino acid like arginine, histidine and Lysine [34]. L-cysteine molecules
in gold colloidal solution are interlinked through sulphur, NH3+ and COO groups [35]. The adsorption of Histidine on gold nanoparticles were analyzed by Soft X-ray photoelectron spectroscopy and near edge X-ray absorption fine structure spectroscopy. Vacuum deposition of thin histidine films on polycrystalline gold was inspected by High-Resolution Synchrotron Photoemission. For monolayer films amino groups, N3 atoms of imidazole rings, and carboxylate groups are probably involved in the bonding to the gold. In the multilayer film histidine form 3D aggregates held with strong intermolecular forces in between them and weakly interact with gold substrate. The equal proportion of protonated amino group and protonated imidazole are existed there. The soft X- ray for multilayer film induces neutral molecules and a mass loss via decarboxylation [36].
Within vacuum chamber the cysteine molecules were evaporated on standard gold {111} single crystal substrate. When this deposited material is analyzed by scanning tunneling microscopy under Ultrahigh Vacuum Conditions, it was observed that at room temperature and sub-monolayer deposition elongated molecular islands having no molecular order with a highly ordered molecular layer of quadratic symmetry are coexist. Upon increasing dosage of deposition structures with short-range hexagonal order were observed. After annealing to 380K drastic change in surface morphology and adsorption structure with superstructure characteristic for alkanethiol self-assembled monolayers on Au- (111) was observed. It was concluded that covalent S-Au bonding takes place by the deposition [37].
First principles quantum mechanical treatment (density functional theory) was performed on interaction of tryptophan- gold interaction [38]. It was found that carboxyl and indole functional groups were involved in the interaction with metal. It depicts interactions along with small ionic interactions instead of van der Waals interactions between Trptophan and Au nanoparticles. Trptophan-Au complex has shown fluorescence quenching in Trptophan by introducing hybrid states associated with Au as low-lying excited states which gives non radiative energy transfer to these states. Gold nanoparticles were synthesized by assisting various amino acids [39]. The details of synthesized method for gold nanoparticles assisted with various amino and pH are given in following table.
Synthesis method |
pH |
Amino acid |
Binding detail |
Each amino acid was mixed with HAuCl4+Filtraion+0.5mol/L NaOH+ stirring in dark 30 minutes +irradiated by UV light |
10 |
L-Glycine L-Aspartate L-Serine L-Leucine lysine |
Gold NPs stabilized Amino acid whose Amine groups are Bound to the NP surfaces |
50 mL solution of 0.64mM of L- Histidine+25.4 mM HAuCl4+3 minutes stirring+ NaOH+150oC for 12hours |
11.50 |
L-Histidine |
Amine group or N atom of imidazole group adsorbed on gold nanoparticles surface |
0.1mM of HAuCl4 prepared in deionised water+heated to boiling+0.25 mM sodium borohydride solution+amino acid(1:10 molar ratio of amino acid to gold solution) |
physiol ogical pH |
L-Arginine L- Glutamic Acid |
electrostatic interaction was observed between gold surface and amine group of L-Arginine at the pH of 7, covalent/coordinate bond observed between N/Au than that of L-Glutamic acid synthesized at pH 3. |
||
Amino acid+10 mL of 0.1 M KOH solution+10 mL solution of 50 mM KAuBr4 added drop-wise |
>7 |
L-tyrosine, glycyl-L- tyrosine, and L- arginine |
— |
||
Deposition in UHV chamber |
— |
S-histidine |
Histidine adsorbs through the depronated carboxylate group in a quasi-upright geometry that maintains the imidazole ring far from the surface |
||
0.01% tetrachloroaurate and 0.5 mg/mL aspartate for 12 h mild stirring at 25oC |
— |
Aspartate |
— |
||
90 ml of 104 M aqueous solution of HAuCl4 reduced by 10 ml of 102 M solution of aspartic acid under boiling condition |
— |
aspartic acid |
— |
||
Heated method: L-Proline solution was added in boiled HAuCl4(8.0mL,3.0mM) under vigorous agitation. After 10 minutes cooled down to room temperature, followed by centrifugation. ambient temperature method:HAuCl4 (8.0 mL, 3.0 mM) was mixed with L-proline solution (2.0 mL, 10.0 M) and stirred vigorously for 48 h at ambient temperature |
— |
L-proline |
— |
||
Thin films of gold nanoparticles (ITO) were immersed in freshly prepared amino acid solution for 18 h |
2 |
L-lysine L-cysteine |
Linkage via amine group |
||
100mL of 10-4 M of HAuCl4+0.01 gm NaBH4 at room temperature +10 mL 10-3 M Lysine+aging 12 hours+centrifuge |
3,7,10 |
Lysine |
At pH 10:linkage of Au to Lysine By -amino group while terminal amine makes H bond with COOH group of neighbor of lysine molecule attached to Au |
Table no.1
AMINO ACID INTERFACED SILVER NANOPARTICLES:
Silver nanoparticles are excellent class of material having antibacterial properties [50, 51]. Therefore, they are preferred to use in the decoration of sweets by thin foil, food packaging. The stability of colloidal solution was found higher in basic pH [52]. The interaction of different amino acids like Cystine Tyrosine, Protine, Glycine, Alanine, Serine, Methionine, Cysteine, Lysine, Arginine, Aspartic acid, Tryptophan with silver ions has been explored [53]. Silver nanoparticles are capable of detecting amino acid like cysteine and cystine
which has sulfur in their backbone [54]. Silver nanoparticles are used for colourimetric sensing of cysteine[55]. Cysteine is a thiol-containing amino acid which has critical importance in rheumatoid arthritis, Parkinsons disease and heart disease can be detected by silver nanoparticles [56]. The biomimetic synthesis of silver nanoparticles has possible by taking advantages of silver binding peptides [57]. The adsorption of biothiols onto the surface of AgNPs has given new strategy for analysis of biothiols and sulfur drugs [58]. Biothiols are adsorbrd on the surface of AgNP by forming a covalent bond.
Cysteine and glutathione has ability to form metalligand cluster [59]. Amino acid like Cysteine has tendency to modify the metal surface (silver) in such a way that Cysteine- silver nanoparticles are dispersed in water [60]. They also showed that the aggregation of cysteine-capped colloidal silver particles is interconnected by Hydrogen bonds. The hydrogen bond was observed to form between amine and carboxylic
acid functional group. Amino acid like glycine (neutral non- polar), L-tyrosine (neutral polar), L-aspartic acid (acidic polar) and L-lysine (basic polar) coated silver nnoparticles exhibits stable and homogeneously dispersed nature. L-tyrosine coated silver nanoparticles have highest catalytic activity for Methylene Blue Reduction [61].
Synthesis method |
pH |
Amino acid |
Binding detail |
Amino acid was mixed with AgNO3+addition of MILIQ water+stirrer with vortex mixer for 5 minutes+exposed to 150W Xe lamp |
Cystine-9.1, Histidine-7.8, Methionine-4.0 Tyrosine-10.6, Tryptophan-9.3 |
tryptophan tyrosine, methionine, cystine, histidine |
— |
0.25mM amino acid+2.5mM KOH+addition of AgNO3.The solution was dialyzed |
Tyr-8.2 Try-7.9 |
l-tyrosine,l-tryptophan |
— |
0.5mM cystine was dissolved in 5 mL Ag nano colloidal solution( prepared by solution plasma method) |
— |
l-cystine |
l-cysteine thiolate adsorbs on the Ag nanoparticle surface |
A solution containing 10-3 M of silver nanoparticles was interacted with 10-1 M, 10-2 M and 10-3 M of glutathione for 10 minutes. The mixtures were then kept on the rotary shaker for 10 min at 120 rpm at room temperature for interaction |
Cysteine glutathione |
thiol groups of cysteine bind to silver surface through ligand exchange reactions.The positive aminno group of cysteine formed salt bridges with carboxylate groups. Carboxylic group in glutamic acid,-NH2 group in Glycine and sulfur atom in the cysteine residue. |
|
Hydrothermal method-Ag (NH3)2OH+ 5 ml of 1% histidine+ vigorous stirred for 10 minutes, and then an appropriate volume of 2 M NaOH solution was added+130 °C for 5 hours |
11 |
Histidine |
imidazole moiety of histidine is bound on the Ag surface and COO group is exposed outward |
90.0 mg of AgNO3 in 500 mL of water was brought to boiling.1% sodium citrate was added with vigorous stirring |
— |
Histidine |
Histidine was binded to Ag by carboxylate group |
Table no.2
Glutamine (Gln) and histidine (His) functionalized silver nanoparticles are used as colorimetric sensor for Hg2+ in water because it provides amino (-NH2) and carboxylic acid (- COOH) binding sites for Hg2+[68]. Histidine modified silver nanoparticles manifests less cytotoxicity and has application in cancer therapy [69]. Lysine-functionalized silver nanoparticles are used for detection of histidine and histidine-tagged proteins[70]. L-Histidine functionalized silver nanoparticles indicates that histidine is adsorbed on the NP surface through the imidazole moiety with the COO- functional group projecting outwards[71]. The complex of silver and
tryptophan are showing pronounced antibacterial activity while the complex of silver and histidine are showing lower antibacterial activity[72].The silver zeolite shows that the antibacterial activity has inhibited by the addition of amino acids like L-cysteine, L-methionine, L-histidine, L-tryptophan [73].
According to group, [74] histidine has adsorbed on silver nanoparticles surface through carboxylate group while through imidazole ring on gold nanoparticles. By the same group from the DFT calculation it was interpreted that the coordination via the carboxylate group was much more stable on Ag than on
Au by 17.4 kcal/mol. It has reported that Glutathione- stabilized silver nanoparticles contain SH group can acts as a colorimetric sensor to probe Ni2+ in water [75]. Serine acts as a reducing agent in synthesis of silver nanoparticles by a microwave irradiation [76]. These nanoparticles are observed to stable for more than 6 months. Basic amino acid like L- lysine or L-arginine is excellent reducing agent for uniform, water soluble silver nanoparticles [77]. At pH 7 from surface enhanced Raman spectroscopy L-arginine links with Ag surface through the guanidinium fragment while at pH 9, L- Arginine links with Ag surface through guanidinium, carboxylate and the aliphatic moieties [78]. Glutamic acid capped silver nanoparticles were synthesized from Silver Nitrate, Glutamic acid and glucose by a chemical reduction method [79]. The presence of glutamic acid screen nanoparticles from agglomeration. The capped nanoparticles make them biocompatible too.
Cysteine has tendency to adsorb on metal surface by three functional groups (- NH2/NH3, COOH/COO or SH/S).Cysteine biomolecules were used as electrode modifier [80, 81, 82]. L-cysteine molecules in silver colloidal solution are attached through amino and carboxylate groups[83]. Immobilized silver nanoparticles are more stable, showing less aggregation ability with less oxidation in aqueous medium as compared to colloidal silver nanoparticles. Arginine modified silver nanoparticles exhibits immobilization of silver nanoparticles on ZnO nanorods. It also displays enhanced antibacterial activity for E.coli and Bacillus-subtilis bacterial cells and no cytotoxic effect on human [84].
AMINO ACID INTERFACED CUPPER NANOPARTICLES:
Histidine interacts with the Cu(110) surface only via the carboxylate group and ring imino (N2) nitrogen observed in the XPS study and annealing at 425K the XPS analysis indicates strong interaction of IM ring with the Cu surface by means of imino Nitrogen[85].Reflection absorption infrared spectroscopy (RAIRS) study confirmed that adsorption proceeds via the carboxylate group (COO-) and via the dehydrogenated nitrogen of the imidazole group[86]. According to this group there are three binding sites for adsorption of Histidine on metal surface: an amino group, carboxylate group and imidazole nitrogen atoms.
On gold, histidine mainly adsorbs through the depronated carboxylate group in a quasi-upright geometry that maintains the imidazole ring far from the surface. On copper, the histidine NH2 and COO groups are involved in the interaction with the surface, with the cycle closer to the surface [87].X-ray absorption and emission spectra study and cluster models under DFT theory has proposed that Glycine molecule at 400K formed binding with both Oxygen and Nitrogen atom to Cu nanoparticles [88].
Screen printed carbon electrode modified by Copper nanoparticles can used for efficient detection of all 20 amino acids [89]. This method of detection of amino acid is more stable than other traditional methods like Raman, NMR or circular dichroism. Amino Acid Chelated Copper Nanoparticles indicates enhanced antibacterial activity against Enterococcus faecalis as compared to copper nanoparticles
[90]. It was investigated that Copper nanoparticles modified carbon paste electrode can employed for detection of L- arginine and L-lysine [91]. In aqueous solution the N of imidazole ring of histidine was able to form stable complexes with Cu (II), Ni (II) and Zn (II) [92]. Barlow et al has systematically explained adsorption of Glycine on Cu (110) and Cu (100) takes place below 290K. While it bonds through Oxygen atom of carboxyl and Nitrogen atom of amino group below 408 K. They have studied Alanine adsorbed on Cu(110), S-norvaline on Cu(110), S-methionine on Cu (110), S-serine on Cu(110) and S-proline on Cu(110)also[93].-
Semiconductor nanoparticles:
The binding and nature of interaction of biomolecules with inorganic surfaces are significant for biological evolution theories [94], biomaterial implants [95] and biosensors [96].
-
Amino acid interfaced TiO2 Nanoparticles: Titanium dioxide is one of the most studied and useful material in variety of fields likes paints, sensors, catalyst and electronics devices. The interaction of biomolecule with TiO2 important in the view of understanding the reaction with implanted titanium metal. Surface spectroscopy clears that glycine was adsorbed on TiO2 surface (110) through carboxylic group [97]. The surface modified with glycine when inspected under scanning tunneling microcopy which evident that glycine has been dissociatively adsorbed on TiO2 surface [98]. The adsorption of glycine, methionine, serine, and cysteine on rutile (100) and (110) surfaces were studied by simulated performed with first principles molecular dynamics. Serine forms bond between a deprotonated hydroxyl and Ti surface while cysteine forms bond between thiol group and Ti surface [99]. The detailed analysis of valence band spectra (VBS) and XPS of glycine adsorbed on TiO2 (110) surface at room temperature has revealed that layers formed by glycine molecule is in polar zwitterionic form [100]. They have investigated that multilayers of glycine adsorbed on TiO2 (110) surface via zwitterionic form through three peaks in VBS at 1) 18.1 eV attributed to C 2s from – carbon atom, 2) 27.6eV attributed to O 2s from carbonyl group 3) 24.5eV attributed to N 2s from NH3+(amino) group. Glycine layers get desorbed when they are faced by photons and remaining layer has C, NH1, NH2, OH and Ti3+surface species.
In connection with this DFT approach was used to investigate the contribution of van der Waals forces in the interaction of Glycine with the TiO2(110) surface [101]. It has proved that dissociative adsorption is more favorable. They have found that van der Waals forces do not affect surface deprotonation. DFT approach was used to get insight of adsorption of proline and glycine on the TiO2 (110) surface [102]. As per this approach the most stable configuration is anionic moiety connecting two surface titanium atoms via the carboxyl oxygen atoms. Amino acid forms hydrogen bonds with the surface.
Owing to amphoteric nature of the TiO2and zwitterionic nature of amino acid the carboxylic group replaces surface OH group on Ti site and connects to Ti [103]. They have analysed XPS of adsorbed amino acid on Ti surface and observed that amino acids are adsorbed at only acidic milieu and no
2
2
adsorption in basic pH. The XPS study of adsorbed amino acid on Ti surface has concluded that adsorption is pH dependent due to amphoteric nature of the TiO2 [104]. Thermal desorption spectroscopy of glycine adsorbed on TiO2 surface has observed that glycine layer doesnt desorb at room temperature [105]. The temperature-programmed desorption (TPD) investigation of surface chemistry and binding of DL- proline on a TiO2 single crystal (001) surface has been carried out [106]. Adsorption of proline leads to creation of surface defects. The core level spectra of C1s is lies at 288.0 eV which is much lower than non-dissociative adsorbed carboxylic acid for Proline adsorbed on TiO2{110} single crystal surface. In addition to this the peaks corresponding to CH2 and C-NH has also found. The core level spectra of N1s shows peak corresponding to NHx species due to C-NH (400eV) and other corresponding to C-NH +(401.5eV). The O 1s spectra corresponding to carboxylate group is clearly seen at 532.5eV. The presence of oxygen defects at the surface zwitterionic proline as less oxygen sites available to facilitate the required proton migration from the hydroxyl group of the amino acid [107].
Adsorption of glycine is not observed on rutile surface of TiO2 because of amino group of glycine forms hydrogen bind to water in solution which weakens the binding to TiO2.This principle is applied to polar solvents like CH3CN and CH3OH.Interaction of organic matter is drived by pH and is insignificant at pH<7.At pH 9 the hydroxylation is drastically reduced and deprotonation of the carboxylic groups occurs [108]. XPS and Temperature Programmed Desorption (TPD) techniques are used to probe the adsorption of glycine on rutile TiO2 (011) single crystal. The peaks of Ti (2p) or O (1s) was observed to attenuate by adsorption. Glycine was observed to form zwitterionic (H3+NCH2COO) with less stability and the dissociated (NH2CH2COO) structure with more stability on TiO2 surface [109].
Glycine adsorbed on the TiO2 surfaces like (110), (100) and
3
3
(001) were monitored by glycine modified AFM tips (atomic Force Microscopy) probe. It is cleared that glycine showed a desorption peak on the TiO2 surfaces and not on mica and pyrite. TiO2 (001) and (100) surfaces shows same adhesion forces and shape of the force curve. Whereas in (110) surface the orientation may influenced by glycine adsorption [110]. The interaction of glycine with the rutile TiO2 (110) surface was examined by High-resolution core-level photoemission and scanned-energy mode photoelectron diffraction (PhD) of the O 1s and N 1s.There is affirmation of zwitterions nature NH +CH2COO- with multilayer deposition, at low coverage only deprotonated glycinate species, NH2CH2COO is present. Multiple-scattering simulations of the O 1s PhD data convey that glycinate is bonded to the surface through the two carboxylate O atoms. The hydrogen atom from deprotonation form hydroxyl species. Non-appearance of major PhD modulations of the N 1s emission is consistent with the amino N atom not being involved in the surface bonding [111].
The first principle calculations were employed to study the adsorption of proline and glycine on anatase (001) surface. The calculated adsorption energy confirms that amino acid strongly interact with (001) surface in the dissociative adsorption manner. This interaction in between oxygen atoms of the amino acid and surface titanium atoms is responsible for
binding of amino acid to TiO2 surface. The dissociative adsorption configuration for proline is having maximum interaction energy of 1.81 and 2.38eV while for glycine maximum interaction energy is 1.53 and 2.11eV [112].Surface oxygen to form more hydrogen bonds with the hydroxyl hydrogen of the biomolecule, therefore L-serine adsorbed on anatase TiO2(101) surface forms biocompatible substrate in benthic microbial fuel cells [113].In the dynamic study of histidine adsorbed in TiO2 nanoparticles by attenuated total reflectance Fourier transform infrared (ATR- FTIR) spectroscopy has ascertained that both the imidazole side chain and the amine group interacting with the TiO2 surface and the adsorption to be reversible [114].
Photodegradation study of L-Alanine adsorbed on TiO2 was performed. This adsorption is observed to possible when l- alanine is zwitterionic and titania surface is positively charged. The pallete of this material was kept under UV light source through quartz wall then it is out gassed followed by recording IR spectrum. It was observed that photo-degradation possible through removal of protonated amino group followed by mineralization of carbon atoms due to ·OH radicals [115].
The amino acids like Serine containing OH group, histidine and tryptophan containing NH and asparagine containing – NH2 in their side chain appeared to adsorb more favorably by the side chain. They are vulnerable to photocatalytic oxidation and showed high decomposition rates. However, alanine having aliphatic side chain interacts with the acidic bridged OH groups on TiO2 [116]. Theoretical study of adsorption of cysteine on TiO2 (110) has been carried out and it was concluded that cysteine interact with TiO2 (110) surface through carboxylic group, the amino and sulfur groups [117]. The systematic X-ray photoelectron spectroscopy investigation was employed in order to understand that mechanism of bonding off L-cysteine molecules on a rutile TiO2 (110) single crystal surface at 65 °C and room temperature. It was clarified that deprotonated carboxylic group forms a double bond with Ti atoms. Thiol groups remain intact with Ti atoms [118].
The infrared studies have observed that when pH of the solution varies in range 2-8, cysteine is adsorbed on TiO2 from aqueous solutions. At pH 2.0, cysteine is adsorbed mainly in protonated and zwitterionic forms, at pH 5.0; cysteine is adsorbed in zwitterionic forms. At pH 8.0, the electrostatic interactions between protonated amine parts and negatively charged TiO2 surface are favored [119].
-
Amino acid interfaced ZnO Nanoparticles: Organic reaction at oxide surfaces has well illustraed for ZnO, TiO2 and other oxide surfaces [120]. With relatively cheaper and biocompatible ZnO has always been benevolent material for application perspectives. The bio-inspired approach when applied to biomolecules like amino acid and inorganic phase nanostructures then the examples of such types are very obvious in nature. Some natural examples are histidine is connected to zinc in zinc finger, configuration of DNA binding protein [121], guanine crystals found in the skin of many fish [122] and many zinc complexes with amino acid [123]. The zinc salts when mineralized from zinc nitrate and hexamethylenetetramine (HMTA) in presence of amino acid then variety of morphologies are formed as per type of amino
acid [124]. The presence of Citrulline, glycyl-glutamine and proline led to form micron zincite crystal, histidine form nano sized crystals, Arginine from nanoscale platelets, Glycyl- glutamine form generates ribbon like coating. Histidine prevents the tendency of ZnO to form larger and elongated crystals. Thus, amino acids molecules are acting as structure directing agent.
Chemicals
pH
Amino acid
Binding
Zn(CH3COO)2 · 2H2O, NaOH, 150
°C for 10 h,
—
histidine
Histidine adsorbed on ZnO surface
0.035 mol zinc acetate dihydrat+
His+ magnetically stirrered+HMT
7
histidine
Polar functional group of his react with Zn2+
Zn(NO3)2,6H2O, NH4OH
—
all
—
0.2 M solution of zinc acetate dihydrate
((CH3COO)2 Zn·2H2O) +0.1 or 0.2M AA
9
l-alanine, l-threonine, l-glutamine
Adsorption of COO on the positively charged (0 0
0 1)-Zn
terminated hexagonal facets
combustion of nitrate- organic fuels
—
L-Glutamine, Leucine ,L- Valine
—
hydrothermal process: zinc acetate dihydrate, a certain amount of amino acid and 6.0 mmol urea:1200C
—
glutamine, histidine and glycine
—
Zn(NO3)2,6H2O:
hydrothermal reaction
—
Lysine
—
Table no.3
The systematic explanation of formation of histidine capped ZnO has observed by considering the ratio of Zn2+ to OH- and Zn2+ to histidine molecule [125]. They have observed that histidine or OH- are binding to Zn2+at different constant values and therefore Zn(OH)n and Zn(C6H9N3O2)n complexes are formed. The variety of morphologies like prism, flower and crystalline hollow microsphere were formed with the presence of L-histidine by varying the ratio of Zinc ion and hydroxide ion [125]. This group has proposed that at the lower concentration of hydroxide ion, histidine molecule is competitive to hydroxide ion to coordinate to Zn2+.As a result of this flower like structure is formed. At the higher concentration of hydroxide ion, Zn(OH)n complexes are formed which is responsible to form anisotropic prism-like structures. The XPS analysis of Nitrogen, Carbon and Oxygen atoms revealed that alanine was found to adsorbed on the non polar surface of ZnO 0) in zwitterionic form [132]. It confirms that carboxylic acid group is linked to ZnO surface. Glutamic acid acts as surface modification agent in ethanol which generates the variation in morphology like twinned
cone, twinned prism. With glycine twin-prism structure with smooth surface, sharp prism and symmetrical sections [133].Aspartic acid, glutamic acid, and alanine are efficient surfactant for ZnO results to various structures[134].Addition of histidine in ZnO suspension inhibits the antifungal acivity against C. albicans as histidine is a quencher of hydroxyl radicals and singlet oxygen [135]. The antibacterial effect of ZnO was observed to decrease by the introduction of histidine for Escherichia coli bacteria [136]. Thus quenching effect is evident by above observation. The influence of ZnO nanoparticles (size < 50nm) on the environment in terms of the reaction between four representative amino acids (Glycine, L-Glutamic, L-Aspartic and L-Histidine) with ZnO nanoparticles has been screened. The increase in the concentration of amino acid has resulted in decreased in particles sized distribution, decreased in percentage zeta potential and reduction in agglomeration [137].
Prism-like and flower-like morphology of ZnO and indium-doped ZnO is obtained by hydrothermal synthesis method with the aid of histidine [138]. Anastasia Brif et al have incorporated various amino acids in ZnO crystal by simple chemical method. They have found that incorporation of different types of amino acid in the crystal induces strain, alters band gap and change in the variation of morphology. Serine and arginine were observed to incorporate to highest level and linear correlation between lattice distortion and band gap alteration has been observed. In connection with this they have found that when amino acid modified ZnO is annealed at 300oC for 90 minutes will decompose the amino acid results to strain and band gap relaxed [127]. Substantial influence on morphology of ZnO has been found by the presence of gelatin in aqueous solution [139]. From the study of binding constant and number of binding sites with Thermodynamic parameters and the nature of binding forces confirms that in the complex of L- tryptophan-ZnO nanoparticles the binding forces are van der Waals interaction and hydrogen bonding [140]. Density Functional Theory (DFT) demonstrates that Arginine and Aspartic acid has good affinity to interact with non-polar surface of ZnO than histidine [141].
The harsh chemical condition and high temperature requirement can avoid by using environmentally friendly material are preferred to use for synthesis. ZnO synthesized by using amine and citrate additives [142]. The tuning of band gap of ZnO can achieve by using histidine acts as capping agents for ZnO nanoparticles [143]. ZnO nanoparticles of 20nm and 70nm treated with L-serine were showing no agglomeration with any alteraration of surface charge [144]. Coating of citrate or L-serine to powder of ZnO nanoparticles induces negative or positive surface charge respectively [145]. The presence of amino acid during the synthesis of ZnO nanoparticles will tailor the band gap via inducing the strain the host crystal [146].
ZnO synthesized in presence of glutamine shows rose-like morphology, in presence of histidine shows dandelion-like morphology and in presence of glycine shows flower-like morphology. Glycine has simpler structure having only 2 carbon atoms leads to loosely assembled structure with larger size; histidine has complex structure having more number of carbon atoms leads to close packed structure with smaller size
[147].The lysine molecules are used as shape directing agent in hydrothermal method; it generates dumbbells, twin-prisms, whiskers, and bundles like morphology for different concentration of lysine. Lysine decorated ZnO exhibits good photocatalytic activity for decomposition of NO under UV-vis light irradiation [148].The unique nest-like ZnO hierarchically porous structures were obtained with the aid of precursors Zinc acetate, glycine, polyvinyl pyrrolidone (PVP), and sodium sulfate [149]. This porous nature has high sensitivity and fast response and recovery speed suggesting potential applications in gas sensing property. For ZnO core shell nanoparticles L-cysteine molecules are serving as a source of sulphur for ZnS shell around ZnO [150]. This makes ZnO nanoparticles more stable in water by cysteine molecules and results in enhancement in zeta potential. Photoluminescence indicates a significant blue shift of 35 nm in near band edge emission with decrease in defect luminescence. The exitence of cysteine makes this material biocompatible also.
Co-precipitation method is employed for synthesis of cysteine capped ZnO/graphene oxide composite. The synthesized composite manifests good photocatalytic activity for degradation of dye RhB [151]. The systematic exploration of control in size of ZnO was achieved by using cysteine molecule with zinc nitrate and HMTA. The pH was kept to 6.3 it produces the hollow amorphous ZnO microspheres [152]. Cysteine functionalized ZnO quantum dots depicts optical absorption in the visible range and good colloidal stability over wide pH range (pH: 2-12). It was interpreted that chemical coordination of the ligands to Zn2+ centers. Which concomitantly reduce the numerical density of oxygen defects on or near the surface [153].
Biomolecules like L-Lysine, L-Cysteine and L-Arginine can engaged to control the shape and ultimately properties of ZnO. The synthesis was carried out with zinc acetate, 0.5 mmol of amino acid and a 100 mL solution of distilled waterethanol (1:1 v/v).The amino acid assisted ZnO in conjunction with multi-walled carbon nano-tube are used as a glucose sensor [154]. Pure Zn substrate was dipped in solution (pH-10) containing Zn2+ and L-cysteine generates 1D vertically aligned ZnO nano-rod array [155]. The steady, time resolved and FT-IR spectroscopy was employed to investigate the specific binding of L-tryptophan with nanoparticles of ZnO. Results derived from both experiments and theoretical computations demonstrate that the ZnO nanoparticles form H- bonding with NH group of amino acid but when both COOH and NH are present in amino acid then ZnO nanoparticles prefer to bind the COOH group rather than the
NH group through H-bonding. The effect of H-bonding is strong in neutral medium (pH~7) while it is weak in basic (pH
~ 10) and acidic (pH ~4) medium [156].
Ag/ZnO nanocomposites were synthesized by solvothermal method in presence of L-arginine. This nanocomposite manifests excellent photocatalytic activity for methyl orange [157].L-arginine modified ZnO indicates enhanced nonlinear refraction and nonlinear absorption with blue shift in excitonic absorption peaks [158]. Biodegradable nanocomposite synthesized from ZnO, starch and lysine can be useful in food packing applications to improve the self life of the food products [159].Paper-like ZnO nanowire films when treated
by l-lysine can enhance the UV photoconduction and suppress the oxygen-vacancy-related photoluminescence [160]. ZnO flowers with nanorods have been synthesized by using lysine as capping agent [161].
Molecular dynamics simulations approach was employed to study the interaction of amino acid and ZnO-water interaction. Glycine exhibits the strongest attraction to (1010) with binding energy 2.57 kJ /mol [162]. The largest binding energy 7kJ/mol was found for tyrosine interacting with the surface in which the Zn ions are at the top. The surface with Zn atoms at the top has binding for all amino acid with 2.60 kJ
/mol whereas the surface with O atoms at the top has non- binding for most amino acid. Self-consistent-charge density- functional-based tight-binding (SCC-DFTB) method was performed on (1010) ZnO Surfaces for glycine adsorption [163]. Glycine molecule binds to the (1010) surface through both functional groups for 0.5 and 0.25 monolayer coverage. Glycine molecule binds to the (1010) surface through the medium of carboxyl or the amine group for one monolayer coverage. Electronic properties of the surface get affected when adsorption occurs through amine group. In all cases the glycine adsorbs dissociatively for all coverages.
Density functional theory clarify that Arginine and Lysine are the preferential binding sequence for ZnO surface with the larger adhesion energy. Arginine is the amino acid with the strongest adhesion on the dry surface, while Lysine is the most stable on the hydrated surface [164]. The adsorption of glycine on zinc-terminated polar (0001) surface has been studied by Density Functional Theory [165]. A study at low coverage glycine adsorbs on ZnO surface preferentially in anionic form with carboxylate moiety forms a ZnOCOZnO with Zn-N bond between amine and Zn surface. The detailed study at high coverage revealed that a glycinate bilayer is formed at the ZnZnO surface. First layer of glycine adopts honeycomb ZnO lattice. In second layer Znglycinate complex are observed in which Zn ions are chelated by glycine through the carboxylic and amine group.
Density functional theory calculations were performed to study site specific interactions between tryptophan and ZnO for tryptophanZnO nanoparticles complex. The calculations demonstrate that COOH group is more actively participate in the bridging than indole and amine groups in tryptophan. The interactions between ZnO and tryptophan were facilitated by ionic and hydrogen bond. These complex exhibits quenching of fluorescence due to non- radiative energy transfer by -*of Tryptophan indole functional group [166].
-
Amino acid interfaced Calcite Mineral:
Calcite occurred in building material in marine organisms. Synthetically it acquires a regular rhombohedra structure along with fracture by cleavage along low energy lattice planes. [167].Calcite (CaCO3) is naturally hierarchical materials and one of the naturally inspired crystalline materials. It existed in the three forms aragonite, minerals calcite and vaterite. Depending on the charge, side chain or nature of amino acid the crystal gets modified [168]. Cysteine, histidine and arginine have a profound inhibition on calcite crystal growth when these amino acids are bound to crystal [169]. This induces the surface covered with the holes. Further
it was observed that the formation of aragonite on crystal substrate was taking place around the holes produced by inhibition.
Owing to different charge and polarity of side chains at applied experimental conditions, L-aspartic acid, L-lysine, L- asparagine, L-tyrosine, L-phenylalanine, L-serine and L- alanine were used for the interaction of this amino acid with calcite crystal at pH 10.76 [170]. The non-polar amino acid has low consequence on structure; morphology whereas it is more pronounced by polar amino acid. L-aspartc acid has most engineered structure, morphology with strong distortion on c-direction. Low concentration of L-aspartic acid leads to formation of vaterite, while at higher concentrations only calcite has been observed. In case of L-lysine, L-asparagine, L-tyrosine and L-serine the formation of additional steps on surfaces are seen.Impact of organic additives like aspartic acid
and glycine on amorphous calcites crystal has performed and [171]. It was shown that the increase in concentration of
window for the tremendous applications in diverse sectors like biomonitered or biocontrolled air-purifier and bio-filter.
REFERENCES:
-
Cao,S.,Wang,Y.,2011. Nanostructures and Nanomaterials: Synthesis, Properties, and Applications, 2nd edn., World Scientific Publishing Company
-
Wang, F., Wang, Y.C., Dou, S., Xiong, M.H., Sun, T.M. and Wang, J., 2011. Doxorubicin-tethered responsive gold nanoparticles facilitate intracellular drug delivery for overcoming multidrug resistance in cancer cells. ACS nano, 5(5), pp.3679-3692.
-
You, C.C., Miranda, O.R., Gider, B., Ghosh, P.S., Kim, I.B., Erdogan, B., Krovi, S.A., Bunz, U.H. and Rotello, V.M., 2007. Detection and identification of proteins using nanoparticle fluorescent polymer chemical nosesensors. Nature nanotechnology, 2(5), p.318.
-
Daniel, M.C. and Astruc, D., 2004. Gold nanoparticles: assembly, supramolecular chemistry, quantum-size-related properties, and applications toward biology, catalysis, and nanotechnology. Chemical reviews, 104(1), pp.293-346.
-
Fedlheim, D.L. and Foss, C.A., 2001. Metal nanoparticles: synthesis,
amino acid the lifetime of crystal. The most influence was observed for aspartic acid and it shows strong binding to calcite. Therefore crysta shows rounded edges with aspartic
-
characterization, and applications. CRC press.
Chen, W., Zhang, J.Z. and Joly, A.G., 2004. Optical properties and potential applications of doped semiconductor nanoparticles. Journal of nanoscience and nanotechnology, 4(8), pp.919-947.
acid and sharp edges with glycine.
The interaction of all amino acid with calcite was explored which demonstrated that cysteine energetically interacts with calcite [172].They have observed that tryptophan has induced maximum distortion followed by phenylalanine in calcite lattice. Controlling crystal structure and morphology of calcite in synthetic material has been observed [173].The amino acid interacts with the calcite in an isotropic manner. Coherence length of amino acid mediated calcite crystal is lower than calcite crystal without amino acid determined from the interpretation of high-resolution synchrotron x-ray radiation [174].The effect of binding amino acids on interfacial energies of growing crystal of calcite has investigated from atomic force microscope, molecular modeling studies ,X-ray photoelectron spectroscopy and surface X-ray diffraction studies[175].The unprecedented finding of increase in hardness with the incorporation of amino acid in calcite crystal was observed [176].
CONCLUSIONS:
The remarkable alterations in the properties of the inorganic matrix material were observed when biomolecules are capped, embedded, adsorbed, incorporated, functionalized or modeled in it. The binding of amino acid to the host matrix are governed by the temperature, pH and type of amino acid. The simple synthesis method, biocompatibility and performance of these organic-inorganic biocomposite are the profound property. The broad spectrum of technological applications in various fields requires proper knowledge of how amino acids attached and detached to inorganic matrix with different parameters. Biomineralization is an approach by which inorganic material is produced in some species. This paves the way to search new engineering between organic and inorganic material to meet new demands in medical and technological field. The substantial efforts of theoretical and practical approach to investigate and understand the the details of the optical and electrical properties of these bio-nano-composites are incredible for the future application in the various fields. These engineered new superior materials have opened a
-
Jaroenworaluck, A., Sunsaneeyametha, W., Kosachan, N. and
Stevens, R., 2006. Characteristics of silicacoated TiO2 and its UV absorption for sunscreen cosmetic applications. Surface and Interface Analysis: An International Journal devoted to the development and application of techniques for the analysis of surfaces, interfaces and thin films, 38(4), pp.473-477.
-
Wang, X., Zhou, J., Song, J., Liu, J., Xu, N. and Wang, Z.L., 2006. Piezoelectric field effect transistor and nanoforce sensor based on a single ZnO nanowire. Nano letters, 6(12), pp.2768-2772.
-
Capper, P., Kasap, S. and Willoughby, A., 2011. Zinc oxide materials for electronic and optoelectronic device applications. John Wiley & Sons.
-
Pileni, M.P., 2003. The role of soft colloidal templates in controlling the size and shape of inorganic nanocrystals. Nature materials, 2(3), pp.145-150.
-
Filankembo, A. and Pileni, M.P., 2000. Is the template of self- colloidal assemblies the only factor that controls nanocrystal shapes?. The Journal of Physical Chemistry B, 104(25), pp.5865- 5868.
-
Hölken, I., Hoppe, M., Mishra, Y.K., Gorb, S.N., Adelung, R. and Baum, M.J., 2016. Complex shaped ZnO nano-and microstructure based polymer composites: mechanically stable and environmentally friendly coatings for potential antifouling applications. Physical Chemistry Chemical Physics, 18(10), pp.7114-7123.
-
Khrenov, V., Klapper, M., Koch, M. and Müllen, K., 2005. Surface functionalized ZnO particles designed for the use in transparent nanocomposites. Macromolecular Chemistry and Physics, 206(1), pp.95-101.
-
Gerstel, P., Hoffmann, R.C., Lipowsky, P., Jeurgens, L.P., Bill, J. and Aldinger, F., 2006. Mineralization from aqueous solutions of zinc salts directed by amino acids and peptides. Chemistry of materials, 18(1), pp.179-186.
-
Qiu, W., Xu, M., Yang, X., Chen, F., Nan, Y. and Chen, H., 2011. Novel hierarchical CdS crystals by an amino acid mediated hydrothermal process. Journal of alloys and compounds, 509(33), pp.8413-8420.
-
Meldrum, F.C. and Colfen, H., 2008. Controlling mineral morphologies and structures in biological and synthetic systems. Chemical reviews, 108(11), pp.4332-4432.
-
Zou, Q. and Yan, X., 2018. Amino Acid Coordinated SelfAssembly. ChemistryA European Journal, 24(4), pp.755-761.
-
Dickerson, M.B., Sandhage, K.H. and Naik, R.R., 2008. Protein-and peptide-directed syntheses of inorganic materials. Chemical reviews, 108(11), pp.4935-4978.
-
Selvakannan, P.R., Mandal, S., Phadtare, S., Gole, A., Pasricha, R., Adyanthaya, S.D. and Sastry, M., 2004. Water-dispersible tryptophan-protected gold nanoparticles prepared by the spontaneous reduction of aqueous chloroaurate ions by the amino acid. Journal of colloid and interface science, 269(1), pp.97-102.
-
Selvakannan, P.R., Swami, A., Srisathiyanarayanan, D., Shirude, P.S., Pasricha, R., Mandale, A.B. and Sastry, M., 2004. Synthesis of aqueous Au core Ag shell nanoparticles using tyrosine as a pH- dependent reducing agent and assembling phase-transferred silver nanoparticles at the air water interface. Langmuir, 20(18), pp.7825- 7836.
-
Si, S. and Mandal, T.K., 2007. pH-controlled reversible assembly of peptide-functionalized gold nanoparticles. Langmuir, 23(1), pp.190- 195.
-
Murphy, C.J., Gole, A.M., Hunyadi, S.E., Stone, J.W., Sisco, P.N., Alkilany, A., Kinard, B.E. and Hankins, P., 2008. Chemical sensing and imaging with metallic nanorods. Chemical Communications, (5), pp.544-557.
-
Ghosh, P., Han, G., De, M., Kim, C.K. and Rotello, V.M., 2008. Gold nanoparticles in delivery applications. Advanced drug delivery reviews, 60(11), pp.1307-1315.
-
Zeng, S., Yong, K.T., Roy, I., Dinh, X.Q., Yu, X. and Luan, F., 2011. A review on functionalized gold nanoparticles for biosensing applications. Plasmonics, 6(3), p.491.
-
Brown, S., Sarikaya, M. and Johnson, E., 2000. A genetic analysis of crystal growth. Journal of molecular biology, 299(3), pp.725-735.
-
Willett, R.L., Baldwin, K.W., West, K.W. and Pfeiffer, L.N., 2005. Differential adhesion of amino acids to inorganic surfaces. Proceedings of the National Academy of Sciences, 102(22), pp.7817-7822.
-
Tan, Y.N., Lee, J.Y. and Wang, D.I., 2010. Uncovering the design rules for peptide synthesis of metal nanoparticles. Journal of the American Chemical Society, 132(16), pp.5677-5686.
-
Shao, Q. and Hall, C.K., 2016. Binding preferences of amino acids for gold nanoparticles: A molecular simulation study. Langmuir, 32(31), pp.7888-7896.
-
Hoefling, M., Iori, F., Corni, S. and Gottschalk, K.E., 2010. Interaction of amino acids with the Au (111) surface: adsorption free energies from molecular dynamics simulations. Langmuir, 26(11), pp.8347-8351.
-
Orza, A., Olenic, L., Pruneanu, S., Pogacean, F. and Biris, A.S., 2010. Morphological and electrical characteristics of amino acidAuNP nanostructured two-dimensional ensembles. Chemical Physics, 373(3), pp.295-299.
-
Zhong, Z., Patskovskyy, S., Bouvrette, P., Luong, J.H. and Gedanken, A., 2004. The surface chemistry of Au colloids and their interactions with functional amino acids. The Journal of Physical Chemistry B, 108(13), pp.4046-4052.
-
Brown, L.O. and Hutchison, J.E., 2001. Formation and electron diffraction studies of ordered 2-D and 3-D superlattices of amine- stabilized gold nanocrystals. The Journal of Physical Chemistry B, 105(37), pp.911-8916.
-
Kasture, M., Sastry, M. and Prasad, B.L.V., 2010. Halide ion controlled shape dependent gold nanoparticle synthesis with tryptophan as reducing agent: Enhanced fluorescent properties and white light emission. Chemical Physics Letters, 484(4-6), pp.271-275.
-
Rawat, K.A. and Kailasa, S.K., 2014. Visual detection of arginine, histidine and lysine using quercetin-functionalized gold nanoparticles. Microchimica Acta, 181(15-16), pp.1917-1929.[35] Jing, C. and Fang, Y., 2007. Experimental (SERS) and theoretical (DFT) studies on the adsorption behaviors of L-cysteine on gold/silver nanoparticles. Chemical physics, 332(1), pp.27-32.
-
Zubavichus, Y., Zharnikov, M., Yang, Y., Fuchs, O., Heske, C., Umbach, E., Tzvetkov, G., Netzer, F.P. and Grunze, M., 2005. Surface chemistry of ultrathin films of histidine on gold as probed by high-resolution synchrotron photoemission. The Journal of Physical Chemistry B, 109(2), pp.884-891.
-
Kühnle, A., Linderoth, T.R., Schunack, M. and Besenbacher, F., 2006. L-cysteine adsorption structures on Au (111) investigated by scanning tunneling microscopy under ultrahigh vacuum conditions. Langmuir, 22(5), pp.2156-2160.
-
Joshi, P., Shewale, V., Pandey, R., Shanker, V., Hussain, S. and Karna, S.P., 2011. Tryptophangold nanoparticle interaction: a first- principles quantum mechanical study. The Journal of Physical Chemistry C, 115(46), pp.22818-22826.
-
Maruyama, T., Fujimoto, Y. and Maekawa, T., 2015. Synthesis of gold nanoparticles using various amino acids. Journal of colloid and interface science, 447, pp.254-257.
-
Cai, H. and Yao, P., 2014. Gold nanoparticles with different amino acid surfaces: Serum albumin adsorption, intracellular uptake and cytotoxicity. Colloids and Surfaces B: Biointerfaces, 123, pp.900- 906.
-
Liu, Z., Zu, Y., Fu, Y., Meng, R., Guo, S., Xing, Z. and Tan, S., 2010. Hydrothermal synthesis of histidine-functionalized single-crystalline gold nanoparticles and their pH-dependent UV absorption characteristic. Colloids and Surfaces B: Biointerfaces, 76(1), pp.311- 316.
-
Zare, D., Akbarzadeh, A., Barkhi, M., Khoshnevisan, K., Bararpour, N., Noruzi, M. and Tabatabaei, M., 2012. L-arginine and L-glutamic acid capped gold nanoparticles at physiological PH: synthesis and characterization using agarose gel electrophoresis. Synthesis and Reactivity in Inorganic, Metal-Organic, and Nano-Metal Chemistry, 42(2), pp.266-272.
-
Bhargava, S.K., Booth, J.M., Agrawal, S., Coloe, P. and Kar, G., 2005. Gold nanoparticle formation during bromoaurate reduction by amino acids. Langmuir, 21(13), pp.5949-5956.
-
Marti, E.M., Quash, A., Methivier, C., Dubot, P. and Pradier, C.M., 2004. Interaction of S-histidine, an amino acid, with copper and gold surfaces, a comparison based on RAIRS analyses. Colloids and Surfaces A: Physicochemical and Engineering Aspects, 249(1-3), pp.85-89.
-
Shao, Y., Jin, Y. and Dong, S., 2004. Synthesis of gold nanoplates by aspartate reduction of gold chloride. Chemical Communications, (9), pp.1104-1105.
-
Mandal, S., Selvakannan, P.R., Phadtare, S., Pasricha, R. and Sastry, M., 2002. Synthesis of a stable gold hydrosol by the reduction of chloroaurate ions by the amino acid, aspartic acid. Journal of Chemical Sciences, 114(5), pp.513-520.
-
Mu, X., Qi, L., Dong, P., Qiao, J., Hou, J., Nie, Z. and Ma, H., 2013. Facile one-pot synthesis of L-proline-stabilized fluorescent gold nanoclusters and its application as sensing probes for serum iron. Biosensors and Bioelectronics, 49, pp.249-255.
-
Orza, A., Olenic, L., Pruneanu, S., Pogacean, F. and Biris, A.S., 2010. Morphological and electrical characteristics of amino acidAuNP nanostructured two-dimensional ensembles. Chemical Physics, 373(3), pp.295-299.
-
Selvakannan, P.R., Mandal, S., Phadtare, S., Pasricha, R. and Sastry, M., 2003. Capping of gold nanoparticles by the amino acid lysine renders them water-dispersible. Langmuir, 19(8), pp.3545-3549.
-
Makarov, V.V., Love, A.J., Sinitsyna, O.V., Makarova, S.S., Yaminsky, I.V., Taliansky, M.E. and Kalinina, N.O., 2014. Green nanotechnologies: synthesis of metal nanoparticles using plants. Acta Naturae, 6(1 (20)).
-
Franci, G., Falanga, A., Galdiero, S., Palomba, L., Rai, M., Morelli,
G. and Galdiero, M., 2015. Silver nanoparticles as potential antibacterial agents. Molecules, 20(5), pp.8856-8874.
-
de Matos, R.A. and Courrol, L.C., 2017. Biocompatible silver nanoparticles prepared with amino acids and a green method. Amino acids, 49(2), pp.379-388.
-
Gruen, L.C., 1975. Interaction of amino acids with silver (I) ions. Biochimica et Biophysica Acta (BBA)-Protein Structure, 386(1), pp.270-274.
-
Athilakshmi, J., Mohan, M. and Chand, D.K., 2013. Selective detection of cysteine/cystine using silver nanoparticles. Tetrahedron Letters, 54(5), pp.427-430.
-
Babu, S., Claville, M.O. and Ghebreyessus, K., 2015. Rapid synthesis of highly stable silver nanoparticles and its application for colourimetric sensing of cysteine. Journal of Experimental Nanoscience, 10(16), pp.1242-1255.
-
Han, C., Xu, K., Liu, Q., Liu, X. and Li, J., 2014. Colorimetric sensing of cysteine using label-free silver nanoparticles. Sensors and Actuators B: Chemical, 202, pp.574-582.
-
Naik, R.R., Stringer, S.J., Agarwal, G., Jones, S.E. and Stone, M.O., 2002. Biomimetic synthesis and patterning of silver nanoparticles. Nature materials, 1(3), pp.169-172.
-
Shrivas, K. and Wu, H.F., 2008. Applications of silver nanoparticles capped with different functional groups as the matrix and affinity probes in surfaceassisted laser desorption/ionization timeofflight and atmospheric pressure matrixassisted laser desorption/ionization ion trap mass spectrometry for rapid analysis of sulfur drugs and biothiols in human urine. Rapid Communications in Mass Spectrometry, 22(18), pp.2863-2872.
-
Brelle, M.C., Zhang, J.Z., Nguyen, L. and Mehra, R.K., 1999. Synthesis and ultrafast study of cysteine-and glutathione-capped Ag2S semiconductor colloidal nanoparticles. The Journal of Physical Chemistry A, 103(49), pp.10194-10201.
-
Mandal, S., Gole, A., Lala, N., Gonnade, R., Ganvir, V. and Sastry, M., 2001. Studies on the reversible aggregation of cysteine-capped colloidal silver particles interconnected via hydrogen bonds. Langmuir, 17(20), pp.6262-6268.
-
Chandra, A. and Singh, M., 2015. Amino Acid Coated Silver Nanoparticles: A Green Catalyst for Methylene Blue Reduction. International Journal of Chemical and Molecular Engineering, 10(1), pp.1-7.
-
de Matos, R.A. and Courrol, L.C., 2017. Biocompatible silver nanoparticles prepared with amino acids and a green method. Amino acids, 49(2), pp.379-388.
-
Shankar, S. and Rhim, J.W., 2015. Amino acid mediated synthesis of silver nanoparticles and preparation of antimicrobial agar/silver nanoparticles composite films. Carbohydrate polymers, 130, pp.353- 363.
-
Tsukada, C., Yagi, S., Nomoto, T., Mizutani, T., Ogawa, S., Nameki, H., Nakanishi, Y., Kutluk, G., Namatame, H. and Taniguchi, M., 2012. Adsorption behavior of l-cysteine on Ag nanoparticles under water environment studied by S K-edge NEXAFS. Applied surface science, 262, pp.231-233.
-
Ravindran, A., Chandrasekaran, N. and Mukherjee, A., 2012. Studies on differential behavior of silver nanoparticles towards thiol containing amino acids. Current Nanoscience, 8(1), pp.141-149.
-
Liu, Z., Xing, Z., Zu, Y., Tan, S., Zhao, L., Zhou, Z. and Sun, T., 2012. Synthesis and characterization of L-histidine capped silver nanoparticles. Materials Science and Engineering: C, 32(4), pp.811- 816.
-
Lim, J.K., Kim, Y., Lee, S.Y. and Joo, S.W., 2008. Spectroscopic analysis of L-histidine adsorbed on gold and silver nanoparticle surfaces investigated by surface-enhanced Raman scatterng. Spectrochimica Acta Part A: Molecular and Biomolecular Spectroscopy, 69(1), pp.286-289.
-
Buduru, P., Reddy, B.S.R. and Naidu, N.V.S., 2017. Functionalization of silver nanoparticles with glutamine and histidine for simple and selective detection of Hg2+ ion in water samples. Sensors and Actuators B: Chemical, 244, pp.972-982.
-
Hu, G., Liang, G., Zhang, W., Jin, W., Zhang, Y., Chen, Q., Cai, Y. and Zhang, W., 2018. Silver nanoparticles with low cytotoxicity: controlled synthesis and surface modification with histidine. Journal of materials science, 53(7), pp.4768-4780.
-
Bae, D.R., Han, W.S., Lim, J.M., Kang, S., Lee, J.Y., Kang, D. and Jung, J.H., 2010. Lysine-functionalized silver nanoparticles for visual detection and separation of histidine and histidine-tagged proteins. Langmuir, 26(3), pp.2181-2185.
-
Nidya, M., Umadevi, M. and Rajkumar, B.J., 2015. Optical and morphological studies of L-histidine functionalised silver nanoparticles synthesised by two different methods. Journal of Experimental Nanoscience, 10(3), pp.167-180.
-
Kazachenko, A.S., Legler, A.V., Per'yanova, O.V. and Vstavskaya, Y.A., 2000. Synthesis and antimicrobial activity of silver complexes with histidine and tryptophan. Pharmaceutical Chemistry Journal, 34(5), pp.257-258.
-
Matsumura, Y., Yoshikata, K., Kunisaki, S.I. and Tsuchido, T., 2003. Mode of bactericidal action of silver zeolite and its comparison with that of silver nitrate. Appl. Environ. Microbiol., 69(7), pp.4278-4281.
-
Lim, J.K., Kim, Y., Lee, S.Y. and Joo, S.W., 2008. Spectroscopic analysis of L-histidine adsorbed on gold and silver nanoparticle surfaces investigated by surface-enhanced Raman scattering. Spectrochimica Acta Part A: Molecular and Biomolecular Spectroscopy, 69(1), pp.286-289.
-
Li, H., Cui, Z. and Han, C., 2009. Glutathione-stabilized silver nanoparticles as colorimetric sensor for Ni2+ ion. Sensors and Actuators B: Chemical, 143(1), pp.87-92.
-
Jayaprakash, N., Vijaya, J.J., Kennedy, L.J., Priadharsini, K. and Palani, P., 2015. Antibacterial activity of silver nanoparticles synthesized from serine. Materials Science and Engineering: C, 49, pp.316-322.
-
Hu, B., Wang, S.B., Wang, K., Zhang, M. and Yu, S.H., 2008. Microwave-assisted rapid facile green synthesis of uniform silver nanoparticles: self-assembly into multilayered films and their optical
properties. The Journal of Physical Chemistry C, 112(30), pp.11169- 11174.
-
Garrido, C., Aguayo, T., Clavijo, E., GómezJeria, J.S. and CamposVallette, M.M., 2013. The effect of the pH on the interaction of Larginine with colloidal silver nanoparticles. A Raman and SERS study. Journal of Raman Spectroscopy, 44(8), pp.1105-1110.
-
Stevanovi, M., Savanovi, I., Uskokovi, V., kapin, S.D., Brako, I., Jovanovi, U. and Uskokovi, D., 2012. A new, simple, green, and one-pot four-component synthesis of bare and poly (, , L-glutamic acid)-capped silver nanoparticles. Colloid and polymer science, 290(3), pp.221-231.
-
Liu, Y., Yuan, R., Chai, Y., Tang, D., Dai, J. and Zhong, X., 2006. Direct electrochemistry of horseradish peroxidase immobilized on gold colloid/cysteine/nafion-modified platinum disk electrode. Sensors and Actuators B: Chemical, 115(1), pp.109-115.
-
Bain, C.D., Troughton, E.B., Tao, Y.T., Evall, J., Whitesides, G.M. and Nuzzo, R.G., 1989. Formation of monolayer films by the spontaneous assembly of organic thiols from solution onto gold. Journal of the American Chemical Society, 111(1), pp.321-335.
-
Ataka, K. and Heberle, J., 2004. Functional vibrational spectroscopy of a cytochrome c monolayer: SEIDAS probes the interaction with different surface-modified electrodes. Journal of the American Chemical Society, 126(30), pp.9445-9457.
-
Jing, C. and Fang, Y., 2007. Experimental (SERS) and theoretical (DFT) studies on the adsorption behaviors of L-cysteine on gold/silver nanoparticles. Chemical physics, 332(1), pp.27-32.
-
Agnihotri, S., Bajaj, G., Mukherji, S. and Mukherji, S., 2015. Arginine-assisted immobilization of silver nanoparticles on ZnO nanorods: an enhanced and reusable antibacterial substrate without human cell cytotoxicity. Nanoscale, 7(16), pp.7415-7429.
-
Feyer, V., Plekan, O., Skala, T., Chab, V., Matolin, V. and Prince, K.C., 2008. The electronic structure and adsorption geometry of L- histidine on Cu (110). The Journal of Physical Chemistry B, 112(43), pp.13655-13660.
-
Marti, E.M., Methivier, C., Dubot, P. and Pradier, C.M., 2003. Adsorption of (S)-histidine on Cu (110) and oxygen-covered Cu (110), a combined Fourier transform reflection absorption infrared spectroscopy and force field calculation study. The Journal of Physical Chemistry B, 107(39), pp.10785-10792.
-
Marti, E.M., Quash, A., Methivier, C., Dubot, P. and Pradier, C.M., 2004. Interaction of S-histidine, an amino acid, with copper and gold surfaces, a comparison based on RAIRS analyses. Colloids and Surfaces A: Physicochemical and Engineering Aspects, 249(1-3), pp.85-89.
-
Nyberg, M., Hasselström, J., Karis, O., Wassdahl, N., Weinelt, M., Nilsson, A. and Pettersson, L.G., 2000. The electronic structure and surface chemistry of glycine adsorbed on Cu (110). The Journal of Chemical Physics, 112(12), pp.5420-5427.
-
Zen, J.M., Hsu, C.T., Kumar, A.S., Lyuu, H.J. and Lin, K.Y., 2004. Amino acid analysis using disposable copper nanoparticle plated electrodes. Analyst, 129(9), pp.841-845.
-
DeAlba-Montero, I., Guajardo-Pacheco, J., Morales-Sánchez, E., Araujo-MartÃnez, R., Loredo-Becerra, G.M., MartÃnez-Castañón, G.A., Ruiz, F. and Compeán Jasso, M.E., 2017. Antimicrobial properties of copper nanoparticles and amino acid chelated copper nanoparticles produced by using a soya extract. Bioinorganic chemistry and applications, 2017.pp.1-7
-
Heli, H., Sattarahmady, N. and Hajjizadeh, M., 2014. Electrocatalytic oxidation and electrochemical detection of guanine, l-arginine and l- lysine at a copper nanoparticles-modified electrode. Analytical Methods, 6(17), pp.6981-6989.
-
Altun, Y. and Koseolu, F., 2005. Stability of copper (II), nickel (II) and zinc (II) binary and ternary complexes of histidine, histamine and glycine in aqueous solution. Journal of solution chemistry, 34(2), pp.213-231.
-
Barlow, S.M. and Raval, R., 2003. Complex organic molecules at metal surfaces: bonding, organisation and chirality. Surface Science Reports, 50(6-8), pp.201-341.
-
Senanayake, S.D. and Idriss, H., 2006. Photocatalysis and the origin of life: Synthesis of nucleoside bases from formamide on TiO2 (001) single surfaces. Proceedings of the National Academy of Sciences, 103(5), pp.1194-1198.
-
Helsen, J.A. and Breme, H.J., 1998. Metals as biomaterials (No. 615.46). Wiley
-
Sorribas, H., Padeste, C. and Tiefenauer, L., 2002. Photolithographic generation of protein micropatterns for neuron culture applications. Biomaterials, 23(3), pp.893-900.
-
Soria, E., Colera, I., Roman, E., Williams, E.M. and de Segovia, J.L., 2000. A study of photon-induced processes with adsorption- desorption of glycine at the TiO2 (110)(1× 2) surface. Surface science, 451(1-3), pp.188-196.
-
Qiu, T. and Barteau, M.A., 2006. STM study of glycine on TiO2
(110) single crystal surfaces. Journal of colloid and interface science, 303(1), pp.229-235.
-
Langel, W. and Menken, L., 2003. Simulation of the interface between titanium oxide and amino acids in solution by first principles MD. Surface Science, 538(1-2), pp.1-9.
-
Soria, E. and Roma, E., 1999. n, EM Williams, JL de Segovia. Surf. Sci, 435, p.543.
-
Tillotson, M.J., Brett, P.M., Bennett, R.A. and Grau-Crespo, R., 2015. Adsorption of organic molecules at the TiO2 (110) surface: the effect of van der Waals interactions. Surface Science, 632, pp.142-153.
-
Tonner, R., 2010. Adsorption of proline and glycine on the TiO2
(110) surface: a density functional theory study. ChemPhysChem, 11(5), pp.1053-1061.
-
Schmidt, M. and Steinemann, S.G., 1991. XPS studies of amino acids adsorbed on titanium dioxide surfaces. Fresenius' journal of analytical chemistry, 341(5-6), pp.412-415.
-
Gold, J.M., 1989. XPS study of amino acid adsorption to titanium surfaces. Helv Phys Acta, 62, pp.246-249.
-
Lausmaa, J., Löfgren, P. and Kasemo, B., 1999. Adsorption and coadsorption of water and glycine on TiO2. Journal of Biomedical Materials Research: An Official Journal of The Society for Biomaterials, The Japanese Society for Biomaterials, and The Australian Society for Biomaterials, 44(3), pp.227-242.
-
Fleming, G.J. and Idriss, H., 2004. Probing the reaction pathways of DL-Proline on TiO2 (001) single crystal surfaces. Langmuir, 20(18), pp.7540-7546.
-
Fleming, G.J., Adib, K., Rodriguez, J.A., Barteau, M.A. and Idriss, H., 2007. Proline adsorption on TiO2 (1 1 0) single crystal surface: A study by high resolution photoelectron spectroscopy. Surface science, 601(24), pp.5726-5731.
-
Ojamäe, L., Aulin, C., Pedersen, H. and Käll, P.O., 2006. IR and quantum-chemical studies of carboxylic acid and glycine adsorption on rutile TiO2 nanoparticles. Journal of colloid and interface science, 296(1), pp.71-78.
-
Wilson, J.N., Dowler, R.M. and Idriss, H., 2011. Adsorption and reaction of glycine on the rutile TiO2 (011) single crystal surface. Surface science, 605(1-2), pp.206-213.
-
Ganbaatar, N., Imai, K., Yano, T.A. and Hara, M., 2017. Surface force analysis of glycine adsorption on different crystal surfaces of titanium dioxide (TiO 2). Nano Convergence, 4(1), p.38.
-
Lerotholi, T.E.J., Kröger, E.A., Knight, M.J., Unterberger, W., Hogan, K., Jackson, D.C., Lamont, C.L. and Woodruff, D.P., 2009. Adsorption structure of glycine on TiO2 (1 1 0): A photoelectron diffraction determination. Surface Science, 603(15), pp.2305-2311.
-
Sowmiya, M. and Senthilkumar, K., 2016. Adsorption of proline, hydroxyproline and glycine on anatase (001) surface: a first-principle study. Theoretical Chemistry Accounts, 135(1), p.12.
-
Zhao, Y.L., Wang, C.H., Zhai, Y., Zhang, R.Q. and Van Hove, M.A., 2014. Selective adsorption of l-serine functional groups on the anatase TiO 2 (101) surface in benthic microbial fuel cells. Physical Chemistry Chemical Physics, 16(38), pp.20806-20817.
-
Mudunkotuwa, I.A. and Grassian, V.H., 2014. Histidine adsorption on TiO2 nanoparticles: an integrated spectroscopic, thermodynamic, and molecular-based approach toward understanding nanobio interactions. Langmuir, 30(29), pp.8751-8760.
-
Martra, G., Horikoshi, S., Anpo, M., Coluccia, S. and Hidaka, H., 2002. FTIR study of adsorption and photodegradation of L–alanine on TiO 2 powder. Research on chemical intermediates, 28(4), pp.359-371.
-
Tran, T.H., Nosaka, A.Y. and Nosaka, Y., 2006. Adsorption and photocatalytic decomposition of amino acids in TiO2 photocatalytic systems. The Journal of Physical Chemistry B, 110(50), pp.25525- 25531.
-
Li, C., Monti, S., Ã…gren, H. and Carravetta, V., 2014. Cysteine on TiO2 (110): a theoretical study by reactive dynamics and photoemission spectra simulation. Langmuir, 30(29), pp.8819-8828.
-
Ataman, E., Isvoranu, C., Knudsen, J., Schulte, K., Andersen, J.N. and Schnadt, J., 2011. Adsorption of L-cysteine on rutile TiO2 (110). Surface science, 605(1-2), pp.179-186.
-
Begonja, S., Rodenas, L.G., Borghi, E.B. and Morando, P.J., 2012. Adsorption of cysteine on TiO2 at different pH values: Surface complexes characterization by FTIR-ATR and Langmuir isotherms analysis. Colloids and Surfaces A: Physicochemical and Engineering Aspects, 403, pp.114-120.
-
Barteau, M.A., 1996. Organic reactions at well-defined oxide surfaces. Chemical reviews, 96(4), pp.1413-1430.
-
Lippard, S.J. and Berg, J.M., 1994. Principles of bioinorganic chemistry (Vol. 70). Mill Valley, CA: University Science Books.
-
LevyLior, A., Shimoni, E., Schwartz, O., GavishRegev, E., Oron, D., Oxford, G., Weiner, S. and Addadi, L., 2010. Guaninebased biogenic photoniccrystal arrays in fish and spiders. Advanced Functional Materials, 20(2), pp.320-329.
-
Rombach, M., Gelinsky, M. and Vahrenkamp, H., 2002. Coordination modes of aminoacids to zinc. Inorganica chimica acta, 334, pp.25-33.
-
Gerstel, P., Hoffmann, R.C., Lipowsky, P., Jeurgens, L.P., Bill, J. and Aldinger, F., 2006. Mineralization from aqueous solutions of zinc salts directed by amino acids and peptides. Chemistry of materials, 18(1), pp.179-186.
-
Wu, Q., Chen, X., Zhang, P., Han, Y., Chen, X., Yan, Y. and Li, S., 2008. Amino acid-assisted synthesis of ZnO hierarchical architectures and their novel photocatalytic activities. Crystal Growth and Design, 8(8), pp.3010-3018.
-
Ma, P., Wu, Y., Fu, Z. and Wang, W., 2012. Fabrication of hierarchical ZnO architectures by a biomineralization process. Advanced Powder Technology, 23(2), pp.170-174.
-
Brif, A., Ankonina, G., Drathen, C. and Pokroy, B., 2014. BioInspired Band Gap Engineering of Zinc Oxide by Intracrystalline Incorporation of Amino Acids. Advanced Materials, 26(3), pp.477- 481.
-
Ramani, M., Ponnusamy, S., Muthamizhchelvan, C. and Marsili, E., 2014. Amino acid-mediated synthesis of zinc oxide nanostructures and evaluation of their facet-dependent antimicrobial activity. Colloids and Surfaces B: Biointerfaces, 117, pp.233-239.
-
Srinatha, N., Kumar, V.D., Nair, K.G.M. and Angadi, B., 2015. The effect of fuel and fuel-oxidizer combinations on ZnO nanoparticles synthesized by solution combustion technique. Advanced Powder Technology, 26(5), pp.1355-1363.
-
Guo, Y., Lin, S., Li, X. and Liu, Y., 2016. Amino acids assisted hydrothermal synthesis of hierarchically structured ZnO with enhanced photocatalytic activities. Applied Surface Science, 384, pp.83-91.
-
Truong, Q.D., Le, T.H., Kimura, T., Yin, S., Sato, T. and Ling, Y.C., 2013. Hierarchical ZnO nanostructures: controlling the synthesis and photocatalytic decomposition of nitrogen monoxide. RSC advances, 3(41), pp.19154-19160.
-
Gao, Y.K., Traeger, F., Shekhah, O., Idriss, H. and Wöll, C., 2009. Probing the interaction of the amino acid alanine with the surface of ZnO (101¯ 0). Journal of colloid and interface science, 338(1), pp.16-21.
-
Liu, H., Huang, B., Wang, Z., Qin, X., Zhang, X., Wei, J., Dai, Y., Wang, P. and Whangbo, M.H., 2010. Amino acid-assisted synthesis of ZnO twin-prisms and functional group's influence on their morphologies. Journal of alloys and compounds, 507(1), pp.326-330.
-
Kim, S.H., Olson, T.Y., Satcher Jr, J.H. and Han, T.Y.J., 2012. Hierarchical ZnO structures templated with amino acid based surfactants. Microporous and Mesoporous Materials, 151, pp.64-69.
-
Lipovsky, A., Nitzan, Y., Gedanken, A. and Lubart, R., 2011. Antifungal activity of ZnO nanoparticlesthe role of ROS mediated cell injury. Nanotechnology, 22(10), p.105101.
-
Dutta, R.K., Nenavathu, B.P., Gangishetty, M.K. and Reddy, A.V.R., 2012. Studies on antibacterial activity of ZnO nanoparticles by ROS induced lipid peroxidation. Colloids and Surfaces B: Biointerfaces, 94, pp.143-150.
-
Molina, R., Al-Salama, Y., Jurkschat, K., Dobson, P.J. and Thompson, I.P., 2011. Potential environmental influence of amino acids on the behavior of ZnO nanoparticles. Chemosphere, 83(4), pp.545-551.
-
Pál, E., Hornok, V., Oszkó, A. and Dékány, I., 2009. Hydrothermal synthesis of prism-like and flower-like ZnO and indium-doped ZnO structures. Colloids and Surfaces A: Physicochemical and Engineering Aspects, 340(1-3), pp.1-9.
-
Bauermann, L.P., del Campo, A., Bill, J. and Aldinger, F., 2006. Heterogeneous nuleation of ZnO using gelatin as the organic matrix. Chemistry of materials, 18(8), pp.2016-2020.
-
Mandal, G., Bhattacharya, S. and Ganguly, T., 2009. Nature of interactions of tryptophan with zinc oxide nanoparticles and L- aspartic acid: a spectroscopic approach. Chemical Physics Letters, 472(1-3), pp.128-133.
-
Singh, S., Singh, B., Sharma, P., Mittal, A., Kumar, S., Saini, G.S.S., Tripathi, S.K., Singh, G. and Kaura, A., 2017. Amino acid functionalized zinc oxide nanostructures for cytotoxicity effect and hemolytic behavior: Theoretical and experimental studies. Materials & Design, 134, pp.10-22.
-
Tomczak, M.M., Gupta, M.K., Drummy, L.F., Rozenzhak, S.M. and Naik, R.R., 2009. Morphological control and assembly of zinc oxide using a biotemplate. Acta Biomaterialia, 5(3), pp.876-882.
-
Yadav, R.S., Mishra, P. and Pandey, A.C., 2010. Tuning the band gap of ZnO nanoparticles by ultrasonic irradiation. Inorganic Materials, 46(2), pp.163-167.
-
Kim, K.M., Kim, T.H., Kim, H.M., Kim, H.J., Gwak, G.H., Paek,
S.M. and Oh, J.M., 2012. Colloidal behaviors of ZnO nanoparticles in various aqueous media. Toxicology and Environmental Health Sciences, 4(2), pp.121-131.
-
Kim, K.M., Choi, M.H., Lee, J.K., Jeong, J., Kim, Y.R., Kim, M.K., Paek, S.M. and Oh, J.M., 2014. Physicochemical properties of surface charge-modified ZnO nanoparticles with different particle sizes. International journal of nanomedicine, 9(Suppl 2), p.41.
-
Brif, A., Bloch, L. and Pokroy, B., 2014. Bio-inspired engineering of a zinc oxide/amino acid composite: synchrotron microstructure study. CrystEngComm, 16(16), pp.3268-3273.
-
Guo, Y., Lin, S., Li, X. and Liu, Y., 2016. Amino acids assisted hydrothermal synthesis of hierarchically structured ZnO with enhanced photocatalytic activities. Applied Surface Science, 384, pp.83-91.
-
Truong, Q.D., Le, T.H., Kimura, T., Yin, S., Sato, T. and Ling, Y.C., 2013. Hierarchical ZnO nanostructures: controlling the synthesis and photocatalytic decomposition of nitrogen monoxide. RSC advances, 3(41), pp.19154-19160.
-
Wang, X., Liu, W., Liu, J., Wang, F., Kong, J., Qiu, S., He, C. and Luan, L., 2012. Synthesis of nestlike ZnO hierarchically porous structures and analysis of their gas sensing properties. ACS applied materials & interfaces, 4(2), pp.817-825.
-
Sandmann, A., Kompch, A., Mackert, V., Liebscher, C.H. and Winterer, M., 2015. Interaction of l-cysteine with ZnO: structure, surface chemistry, and optical properties. Langmuir, 31(21), pp.5701- 5711.
-
Selvin, S.S.P., Radhika, N., Borang, O., Lydia, I.S. and Merlin, J.P., 2017. Visible light driven photodegradation of Rhodamine B using cysteine capped ZnO/GO nanocomposite as photocatalyst. Journal of Materials Science: Materials in Electronics, 28(9), pp.6722-6730.
-
Weinzierl, D., Touraud, D., Lecker, A., Pfitzner, A. and Kunz, W., 2008. Controlled preparation of hollow zinc oxide microspheres from aqueous solution using hexamethylenetetramine and cysteine. Materials Research Bulletin, 43(1), pp.62-67.
-
Arslan, O., Singh, A.P., Belkoura, L. and Mathur, S., 2013. Cysteine- functionalized zwitterionic ZnO quantum dots. Journal of Materials Research, 28(14), pp.1947-1954.
-
Tarlani, A., Fallah, M., Lotfi, B., Khazraei, A., Golsanamlou, S., Muzart, J. and Mirza-Aghayan, M., 2015. New ZnO nanostructures as non-enzymatic glucose biosensors. Biosensors and Bioelectronics, 67, pp.601-607.
-
Liu, L., Fu, L., Liu, Y., Liu, Y., Jiang, P., Liu, S., Gao, M. and Tang, Z., 2009. Bioinspired synthesis of vertically aligned ZnO nanorod arrays: Toward greener chemistry. Crystal growth & design, 9(11), pp.4793-4796.
-
Mandal, G., Bhattacharya, S., Chowdhury, J. and Ganguly, T., 2010. Mode of anchoring of ZnO nanoparticles to molecules having both COOH andNH functionalities. Journal of Molecular Structure, 964(1-3), pp.9-17.
-
Chen, Y.C., Cheng, J., Cheng, J. and Cheng, S., 2015. L-Arginine assisted preparation of Ag/ZnO nanocomposites with enhanced photocatalytic performance. Journal of Materials Science: Materials in Electronics, 26(5), pp.2775-2781.
-
Tamgadge, Y., Pahurkar, V., Sunatkari, A., Talwatkar, S. and Muley, G., 2016, April. ThermoOptical Properties of Amino Acid Modified
ZnOPVA Colloidal Suspension Under CW Laser Illumination. In Macromolecular Symposia (Vol. 362, No. 1, pp. 73-81).
-
Kotharangannagari, V.K. and Krishnan, K., 2016. Biodegradable hybrid nanocomposites of starch/lysine and ZnO nanoparticles with shape memory properties. Materials & design, 109, pp.590-595.
-
Liu, J., Park, J., Park, K.H., Ahn, Y., Park, J.Y., Koh, K.H. and Lee, S., 2010. Enhanced photoconduction of free-standing ZnO nanowire films by L-lysine treatment. Nanotechnology, 21(48), p.485504.
-
Subramanian, N. and Al Ghaferi, A., 2013. A green synthetic route for zinc oxide nanoarchitectures using l-lysine. Materials Letters, 92, pp.361-364.
-
Nawrocki, G. and Cieplak, M., 2013. Amino acids and proteins at ZnOwater interfaces in molecular dynamics simulations. Physical Chemistry Chemical Physics, 15(32), pp.13628-13636.
-
DomÃnguez, A., Moreira, N.H., Dolgonos, G., Frauenheim, T. and Da Rosa, A.L., 2011. Glycine adsorption on (1010) ZnO surfaces. The Journal of Physical Chemistry C, 115(14), pp.6491-6495.
-
Buonocore, F., Arcangeli, C., Gala, F., Zollo, G. and Celino, M., 2015. Adsorption of modified arg, lys, asp, and gln to dry and hydrated ZnO surface: a density functional theory study. The Journal of Physical Chemistry B, 119(35), pp.11791-11797.
-
Irrera, S., Costa, D. and Marcus, P., 2009. DFT periodic study of adsorption of glycine on the (0 0 0 1) surface of zinc terminated ZnO. Journal of Molecular Structure: Theochem, 903(1-3), pp.49-58.
-
Joshi, P., Shewale, V., Pandey, R., Shanker, V., Hussain, S. and Karna, S.P., 2011. Site specific interaction between ZnO nanoparticles and tryptophan: a first principles quantum mechanical study. Physical Chemistry Chemical Physics, 13(2), pp.476-479.
-
Schenk, A.S. and Kim, Y.Y., 2015. Unraveling the internal microstructure of biogenic and bioinspired calcite single crystals. MRS Bulletin, 40(6), pp.499-508.
-
Jackson, T.A. and Bischoff, J.L., 1971. The influence of amino acids on the kinetics of the recrystallization of aragonite to calcite. The Journal of Geology, 79(4), pp.493-497.
-
Mann, K., Siedler, F., Treccani, L., Heinemann, F. and Fritz, M., 2007. Perlinhibin, a cysteine-, histidine-, and arginine-rich miniprotein from abalone (Haliotis laevigata) nacre, inhibits in vitro calcium carbonate crystallization. Biophysical journal, 93(4), pp.1246-1254.
-
-
-
Tobler, D.J., Blanco, J.R., Dideriksen, K., Sand, K.K., Bovet, N., Benning, L.G. and Stipp, S.L.S., 2014. The effect of aspartic acid and glycine on amorphous calcium carbonate (ACC) structure, stability and crystallization. Procedia Earth and Planetary Science, 10, pp.143-148.
-
Borukhin, S., Bloch, L., Radlauer, T., Hill, A.H., Fitch, A.N. and Pokroy, B., 2012. Screening the incorporation of amino acids into an inorganic crystalline host: the case of calcite. Advanced Functional Materials, 22(20), pp.4216-4224.
-
Berman, A., Hanson, J., Leiserowitz, L., Koetzle, T.F., Weiner, S. and Addadi, L., 1993. Crystal-protein interactions: controlled anisotropic changes in crystal microtexture. The Journal of Physical Chemistry, 97(19), pp.5162-5170.
-
Berman, A., Hanson, J., Leiserowitz, L., Koetzle, T.F., Weiner, S. and Addadi, L., 1993. iological control of crystal texture: a widespread strategy for adapting crystal properties to function. Science, 259(5096), pp.776-779.
-
Orme, C.A., Noy, A., Wierzbicki, A., McBride, M.T., Grantham, M., Teng, H.H., Dove, P.M. and DeYoreo, J.J., 2001. Formation of chiral morphologies through selective binding of amino acids to calcite surface steps. Nature, 411(6839), pp.775-779.
-
Kim, Y.Y., Carloni, J.D., Demarchi, B., Sparks, D., Reid, D.G., Kunitake, M.E., Tang, C.C., Duer, M.J., Freeman, C.L., Pokroy, B. and Penkman, K., 2016. Tuning hardness in calcite by incorporation of amino acids. Nature materials, 15(8), pp.903-910.