
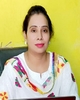
- Open Access
- Authors : Rashmi Sharma , Manendra Mehta , G. Nag Bhargavi
- Paper ID : IJERTV12IS010047
- Volume & Issue : Volume 12, Issue 01 (January 2023)
- Published (First Online): 19-01-2023
- ISSN (Online) : 2278-0181
- Publisher Name : IJERT
- License:
This work is licensed under a Creative Commons Attribution 4.0 International License
Thermoluminescence Properties of Borate Based Phosphors: A Review
Rashmi Sharma Department of Physics Govt. Model College, Atari Raipur (C.G,), India
Manendra Mehta
Department of Physics Govt.E.R.R.PG. Science College Bilaspur (C.G.), India
-
Nag Bhargavi
Department of Physics
Govt. Pt. Shyamacharan Shukla College Dharsiwa Raipur (C.G.), India
Abstract-Thermoluminescence (TL) is the thermally stimulated emission of light from an insulator or a semiconductor following the previous absorption of energy from ionizing radiation. TL dosimetry is used in many scientific and applied fields such as radiation protection, radiotherapy, industry, and environmental and space research, using many different materials. In this article we have discussed some past developments and future opportunities of Borate-based phosphors and their thermoluminescence dosimetry (TLD) properties. For this, we have studied and discussed the synthesis of different borate-based phosphors and the characteristics of TL such as glow curve, dose response, annealing condition, TL sensitivity, fading and reproducibility. The sensitivity of thermoluminescence (TL) phosphors can be increased by the addition of different dopants, co-dopants, rare earth ions or transition metal ions. These doped TLDs are prepared using different techniques including solidstate reaction methods, combustion methods, wet chemical methods, and sol gel methods. The various TLD materials can be irradiated by different types of beams such as rays, Xrays, electrons, neutrons etc. In recent years researchers have given more attention on the new synthesis methods and measurement techniques, still there is a scope for the improvement in the materials properties for TL dosimetry.
Keywords- TLD, Borate based phosphors, Dose response, glow curve.
-
INTRODUCTION
Luminescence is the spontaneous emission of light by a substance not resulting from heat or cold-light, and thus it is a form of coldbody radiation. It can be generated either by electrical energy, chemical reactions, subatomic motions, or stress on a crystal, where incandescence is a light emitted by a substance or object due to heat treatment, this distinguishes luminescence from incandescence. A number of energy conversion processes are possible, by the absorption of photons or charged particles as illustrated in fig. 1, which involve luminescence (photon emission), electron emission, thermal emission, and chemical/structural change.
Fig. 1 – Conversion of primary excitation energy in solids adopted from [1]
In 1565, luminescence was first observed by Monardes in an extract of Lignum nephriticum and fully described by Sir G. G. Stokes as the theoretical basis for the mechanism of absorption (excitation) and emission in 1852. Depending upon the excitation process, luminescence can be classified as photoluminescence, electroluminescence, cathodoluminescence,
chemiluminescence, thermoluminescence, etc. Luminescence can also be classified based on the characteristic time scale () between the excitation and emission of radiation into two parts:
-
Fluorescence where c < 10-8 s (temperature-independent process), and
-
Phosphorescence where c > 10-8 s (temperature-dependent process).
The fluorescence emission simultaneously takes place with the absorption of energy by atoms or molecules followed by immediate emission of light or electromagnetic radiation and suddenly stopped on the removal of the source of excitation. Thus, fluorescence emission is seen to be spontaneous and excited atom has a comparatively short lifetime, c < 10-8 s. On the other hand, phosphorescence is the absorption of energy by atoms or molecules characterized by delayed emission of electromagnetic radiation, and also phosphorescence is seen to continue for some time even after the removal of the source of excitation. The phosphorescence can be further classified into two parts: (a) short period c < 10-4 s (b) and the long period where c >10-4 s, which is called thermoluminescence (TL). Each luminescence process has its significance and benefits as it is one of the rapidly growing and most useful analytical techniques in science and technology. It can be used in a wide range of applications such as materials science, environmental science, microelectronics, physics, chemistry, biology, biochemistry, medicine, pharmaceutical science [2].
Thermoluminescence (TL) is the emission of light from a semiconductor or an insulator when heated following the previous absorption of energy during irradiation [3 5]. In 1889 while studying the classification of luminescence, the term Thermoluminescence was first used by Eilhard Wiedemann. On October 28, 1663, the first official report on the discovery of thermoluminescence was given by Robert Boyle to the royal society of London, where he described TL as a glimmering light and observed from heating diamond in the dark [6]. The TL substance irradiated with cathode rays was first detected by Wiedemann and Schmidt [7] and also reported about the TL activators such as manganese, iron, and rare earth elements systematically [8 – 9].
The thermoluminescence (TL) that could reproduce from the laboratory of natural specimens, was first reported by Trowbridge and Burbank [10]. In 1904, Marie Curie [11] noted that when radium was exposed to the crystal, its TL properties could be restored [12]. The effect of X-rays was tested on the TL of several substances during 19251928 by Wick and co- workers [13 – 14]. During 1925 1930, the TL measurements were done in the form of a glow curve for the first time in Przibrams laboratory [15]. The first controlled and extensive investigation of the first, second, and general order kinetic equation of the thermoluminescence process has been specified by Randall and Wilkins [16], Garlick- Gibson [17], and May- Patridge [18] respectively recorded TL glow curves of several materials and, investigated their trap parameters such as activation energies and frequency factors. The materials must fulfill the following three conditions to get TL emission. Firstly, the material must be an insulator or a semiconductor. Secondly, the material should have some time-absorbed energy during radiation exposure. Thirdly, heating the materials triggers luminescent emission. In the past few years, the researcher has given more attention to the borate based TLD materials due to its some unique properties such as ease of dosimeter handling and preparing, linear dose-response, good thermal stability, low melting point, higher sensitivity, and greater accuracy [19] and to enhance their TL properties, different dopant, co-dopant, activators or rare-earth, were added to the host matrix [20 – 21]. In this article, we have reviewed the various borate-based TLD phosphors and their significant characteristics such as glow curve structure, dose-response, sensitivity, fading, annealing, and reusability which still have better prospects to be utilized further for medical applications, radiation, personal, environmental, and space dosimetry.
-
-
MECHANISM OF THERMOLUMINESCENCE (TL)
Thermoluminescence is the phenomenon process of light emission when the material is the heated charge carrier trap induced by activator impurities in an irradiated thermoluminescent (TL) [22-23]. When materials are exposed to radiation, some bound electrons of an atom forming the crystal lattice become freely mobile and come into the conduction band. Structural defects in the lattice (vacancies, interstitial atoms, and substitutional impurities) created a shortage f localized charge, which act as traps T for the conduction electrons [24]. Most electrons recombine, but some are trapped at deep traps and remain there over geological timescales. At that moment, the charge-deficient ion which contributed to the trapped charge becomes a luminescence center L. Trapped electrons cannot escape from those traps until activation energy is applied. Heat or light can eject charge carriers from traps, and electrons can go back to the conduction band. When these electrons recombine with a luminescence center L and a photon is emitted, hence the process forms the basis of the thermoluminescence and optical dating [25 – 26].
Fig. 2 – Mechanism of electron trapping in the TL process
For simplicity, if we assume that the TL process occurs due to the thermal release of the electron from a single type of trap at a depth E below the conduction band, then the probability of escaping of a single electron per second exponentially depends upon the temperature and is given by
P = s exp (-E/KT)
where s is the frequency factor, E is the activation energy, k is the Boltzmanns constant and T is the absolute temperature.
Thus, the essential condition for the phenomenon of thermoluminescence (TL) [24] in a material is the presence of appropriate defect centers. Defect centers and thermal treatments like heating, the materials at a high temperature, and quenching may be created by doping with suitable impurities. The element added as a dopant to the crystal structure is known as an activator ion, and phosphors that contain activator ions are the most common type [27]. In addition to the activators ions, some more ions may be co-doped into the host lattice as sensitizers (or coactivators), which gather the energy from the exciting source and transfer it to the activator ions. Usually, the luminescence center is nothing but are the activator center. Thus, the luminescent centers are point defects or single activator atoms or groups of atoms, called activators, positioned in the lattice of the host material and serve as discrete centers for localized absorption of excitation energy. Usually, the luminescence center is nothing but are the activator center. Thus, the luminescent centers are point defects or single activator atoms or groups of atoms, called activators, positioned in the lattice of the host material and serve as discrete centers for localized absorption of excitation energy.
-
SYNTHESIS TECHNIQUE FOR THE PREPARATION OF BORATE BASED PHOSPHORS
A, – Combustion Method
Combustion synthesis is an effective method, ecofriendly, and low-cost method to prepare high-purity, small-sized, and spherical particle phosphors. In this synthesis method salts, such as nitrates, metal sulfates, and carbonates, as oxidants and reducing reagents, fuels such as glycine, sucrose, urea, or other water-soluble carbohydrates have been used. During the combustion process, nitrates act as an oxidizer for the fuel. The stoichiometric amount of the starting raw materials is homogeneously mixed and the high temperature is generated instantly by the exothermic reaction that can volatilize low boiling point impurities leading to purer and final products.
The starting raw materials are heated rapidly at 500 – 600 ºC, the solution containing the stoichiometric amount of redox mixture boils, and dehydrates, followed by decomposition, generating combustible gases in a few minutes. The volatile combustible gases ignite, burn with a flame, and a large number of escaping gases dissipates heat, which prevents the material from sintering and thus provides conditions for the formation of the nanocrystalline phase. Also, the generation of gases helps in resulting in the voluminous, foamy, fluppy, and crystalline fine powder occupying the entire volume of the container and has no chance of forming agglomerations unlike in the other conventional processes [28-31]. As reported earlier by Patil et al. this is a versatile method for the synthesis of a wide range of particles, including nanometer-size powder [32].
Advantages: Production of high purity products and homogeneous oxides powder. It is a relatively quick and simple process and saves time and energy. It is useful in many industrial fields such as energy conversion, storage, optical devices, catalysis, electronics, and biomedical applications. In addition to this, the combustion synthesis method has a wide range of applications in different.
Disadvantages: The presence of a relatively huge amount of carbon in the final product. In the combustion method, it is not always easy to define the optimal F/O ratio.
-
– Solid state method
The solid-state method (SSR) is a commonly adopted synthesis route used to produce many kinds of phosphors powders including borates phosphors [33-36]. The sequential steps involved in this method are matter diffusion at interfaces between solid particles, chemical reactions at the atomic level, nuclei formation, and solid-phase transport and growth of a new phase. The solid-state reaction can proceed smoothly and effectively, only when the starting powders have high chemical reactivity, large surface area, good dispersion, or low agglomeration [37].
The solid-state reaction route is the most widely used method for producing multi-component oxides/carbonates/oxalates/nitrates, which involves mixing, grinding, calcination, compacting, and sintering. As solids do not react with each other at room temperature, so for the proper reaction to occur at an appreciable rate, it is required to heat them at higher temperatures, as high as 1000 ºC to 1500 ºC. The major parameters on which the feasibility and rate of a solid-state reaction depend are reaction conditions, the surface area of the solids structural properties of the reactants, their reactivity, and the thermodynamic free energy change associated with the reaction [3839]. For powder processing, a stoichiometric amount of compounds are calculated by taking the ratio of atomic weight and then weighed on a digital balance with greater accuracy. Starting materials are mixed thoroughly using an agate mortar and pestle and are ground into a fine powder for 4 – 5 hours for getting a homogeneous compound. For the subsequent reaction at high temperatures, it is essential to choose a suitable container that is chemically inert to the reactants under heating conditions [40]. The heating conditions depend greatly on the types of the material and reactivity of the reactants. A good furnace is required for adequate heat treatment. There are several conditions under which a solid-state reaction can take place. This synthesis route is very easy, simple, and does not require expensive as well as sophisticated equipment for processing.
Advantages: The main advantage of the SSR method is that the final product is in solid form, which is structurally pure with the desired properties depending on the final sintering temperatures. This method is environmentally friendly and non-toxic. The powders produced from this method are very fine, as well as the cross-contamination is less. This method is also convenient for large-scale production on an industrial scale. This process is low costs simple and easy to handle.
Disadvantages: High temperatures are generally required (500-2000°C) for this method which takes a significant amount of energy to overcome the lattice energy, so there may be a possibility that cation or anion can diffuse into a different site.The reaction may begin very slowly, but increasing the temperature speeds up the reaction since it increases the diffusion rate. Generally, solids are not elevated to their melting point, so reactions take place in the solid-state (subsolidus).
-
– Co-precipitation method
Co-precipitation is the process of letting down by a precipitate of substances normally soluble under the conditions employed [41]. A stoichiometric mixture of soluble salts of the metal is taken and precipitated as hydroxides, citrates, oxalates, or formates. After thn the mixture is filtered, dried, and heated to give the final product. The three main mechanisms of the co- precipitation processes are: inclusion, occlusion, and adsorption [42]. An inclusion (incorporation in the crystal lattice) occurs when the impurity occupies a lattice site in the crystal structure of the carrier, resulting in a crystallographic defect; this can happen until the ionic radius and charge of the impurity are similar to that of the crystal structure of the carrier. Occlusion arises when an adsorbed impurity gets physically trapped inside the crystal as it grows. An adsorbate is the type of impurity that is weakly or strongly bound to the surface of the precipitate.
Advantages: The coprecipitation method has the advantage of directly obtaining pure and homogeneous nanomaterials with small size and size distribution, as compared to the other synthesis process. It is probably the simplest and most convenient technique. There is no wastage of solid. It is an effective and proven technology.
Disadvantage: It is an inexpensive technique. It is difficult to prepare high purity and accurate stoichiometric phases. This technique required a trained person for maintenance and regeneration.
-
– Solgel method
The sol-gel process is a wet chemical method for the synthesis of various nanostructures, especially metal oxide nanoparticles. Firstly, the concentrated or colloidal solution of the reactants, the sol is prepared and then further concentrated to form the gel. Sol is a colloidal suspension of particles in a liquid (~1-100 nm diameter), and a gel is a semi-rigid solid in which the solvent is contained in a framework of material that is either colloidal or polymeric. The molecular precursor is dissolved in water or alcohol by hydrolysis/alcoholysis and converted to gel by dehydrating or polymerizing. The gel obtained is wet, it should be dried using appropriate methods depending on the desired properties and application of the gel. For example, if it is an alcoholic solution, the drying process is completed by burning alcohol. After the drying process, the gels obtained are powdered and calcined. As the sol-gel process involves changing the state from sol to gel using a variety of techniques, mostly the drying process is used to remove the solvent [43-46].
This method is cost-effective and used for making ceramics as a molding material and also used as an intermediate between thin films of metal oxides in various applications. This method has good control over the chemical composition of the products due to the low reaction temperature. The sol-gel method can be used in the process of making ceramics as a molding material and can be used as an intermediate between thin films of metal oxides in various applications. The product obtained from the sol-gel method is used in various fields, such as optical, electronic, energy, surface engineering, biosensors, and pharmaceutical and separation technologies. In addition, with the help of this method, it is possible to make highly homogeneous composites with very high purity (99.99% purity)
Advantages: It is a simple and efficient process. The obtained product has good uniformity and high purity. It can design and control chemical composition and is used to prepare coatings, fibers, and composite materials. It is an energy-saving method due to the low annealing temperature.
Disadvantages: The cost of the raw materials may be high. Duration process may be long. There is often a large volume shrinkage and cracking during drying
-
-
DETERMINATION OF TL PARAMETERS
The dosimetric properties of thermoluminescence materials generally depend on the kinetic parameters such as trap depth, frequency factor, and order of kinetics [47]. These parameters give valuable information about the TL mechanism of phosphors and stability of the traps and show whether the trapped charge carriers will be retrapped on heating or not. If the trap depth is low, then the glow peak appears at a relatively lower temperature, and the corresponding trap is unstable. Due to this, fading of the TL signal occurs at ambient temperature, and fading may be pronounced if the frequency factor is high. Several methods have been proposed for the determination of TL parameters and also explain the mechanism of thermoluminescence among these methods are the initial rise method, peak shape method, and variable heating rate method.
-
– Symmetry factor and order of kinetics
The first, second, and general order kinetic equations governing the thermoluminescence processes and describing the position, shape, and intensity of TL peak have been given by Randall – Wilkins [16], Garlick – Gibson [17], and May – Patridge [18] respectively.
First Order Kinetics – Randall and Wilkins explained the first-order kinetics equation of the TL process in the simplest way [48- 49]. According to this, the existence of the TL peak is due to the thermal release of electrons from traps and their subsequent recombination with holes trapped in the recombination centers. Some assumptions depend on the fact that the rate of recombination is equal to the rate of thermal release of the electron. These assumptions are (1) Only one trapping state and one recombination center are affected, (2) Electrons are thermally released from traps and quickly recombine with trapped holes, and (3) Accumulation of an electron in the conduction band is assumed to be impossible.
This rate of release is proportional to the concentration of trapped electrons n and Boltzmann exponential factor exp at a given temperature (T). Based on these assumptions, the first-order kinetic equation is given by -.
= = [/] (1)
A negative sign indicates that n(t) decreases with the increase of time. By assuming a heating function, T = T(t), we can obtain
an expression for 'n' as a function of temperature. For this, considering the simple linear heating function,
T = To + t (2)
Where, To is the initial temperature and is the heating rate. Now, n(t) can also be expressed as,
() = 0 [ ( 0 () ] .(3)
So, the temperature dependence of TL intensity is given by,
() = 0 ( ) [( ) 0 () ] .(4)
The first exponential term dominates when T is slightly above To, due to which the glow curve increases from its initial
condition and the second exponential term dominates at the higher temperature region. The salient feature of the first-order kinetics is that the dosimetric peak is asymmetric it decreases at high temperatures above the maximum limit and is faster than the increases at low temperatures. Also, the shape of the curve does not change due to changes in the initial concentration.
Second order Kinetics – Garlick and Gibson [17] discussed the second-order kinetics for one trap and one recombination center model. It has two assumptions: (1) The free charge carriers have an equal probability for retrapping and recombination. (2) During the entire process, the concentration of electrons in traps and holes in the recombination center are equal. Consider a case where the total number of traps as given is N then the kinetic equation is given by
= = (
) 2 ( ) ..(5)
Where S/N has the units of m3/s and denoted by S. Hence,
= = 2 exp ( ) ..(6)
Again, consider a linear heating function as same as first order kinetics and after solving second order partial differential
equation we get,
2
0
2
() = 0 exp ( ) [1 + ( ) 0 exp (/ ) ]
..(7)
The salient feature of this equation is that the glow curve is nearly symmetric with the high-temperature half of the curve
slightly wider than the low-temperature half. As identical to the first-order kinetic, the initial rie of the glow curve is dominated by the exponential term exp(-E/KT). The second term dominates at high temperatures hence the product function also decreases, and with the increase in initial concentration, the peak shifts towards the higher temperature. Before they combine there is a
slight spreading of the TL emission on the high-temperature side due to the retrapping of the significant concentration of the released electrons which causes a delay in TL [50].
General Order Kinetics – May and Patridge [18] first suggested an empirical relation for the general order kinetic of the TL given by:
I = nbsexp(-E/KT) .(8)
Where s has the dimensions of m3(b-1) s-1 and b is the order of kinetics which need not necessarily be 1 or 2.
The main difficulty with the empirical equation is understanding the physical meaning of the parameter s, whose dimensions vary with the order of kinetics. This problem was resolved by Rasheedy [51] who modified the equation (8) to the form,
= = (
) exp ( ) ..(9)
1
This equation can be reducing to first order kinetic equation (1) for b = 1 and second order kinetic equation (5) for b = 2.
Integrating equation (9), we get
1
(
(1) 0 )1
= 0 exp ( )
[1 +exp ( ) ]
0
1(10)
The equation (10) is also a empirical relation, which removes the difficulty in understanding the meaning of parameter s.
-
Initial rise method
Garlick and Gibson suggested the simplest method to determine the trap depth of TL materials and is independent of the order of kinetics [17]. It is based on the assumption that for T Tm and I Im, the number of trapped electrons is approximately constant at the rising part of the glow curve, and the dependence of n(T) on temperature T is negligible.
The TL emission can be written as I (t) exp (-/KT) ..(11) Where Tm is the glow peak temperature and Im is the peak intensity.
So, the plot of the logarithm of intensity against 1/T is a straight line with a slope proportional to (-E/K) and with the help of this trap depth can be calculated.
-
– Peak shape method
The Tl glow peaks corresponding to the second-order kinetics are almost symmetrical in shape, whereas those corresponding to the first-order kinetics are asymmetrical. Hence from the glowing shape and geometrical properties of the glow peak, one can get information about the trap depth, frequency factor, and order of kinetics by utilizing peak temperature (TM) and two temperatures corresponding to the half peak intensity on the rising end (T1) and falling end of the glow curve (T2). The total peak width at half maximum intensity ( = T2 T1), the lower temperature half-width ( = TM T1 ), and the upper-temperature half-width ( = T2 TM ) were calculated with the help of glow curve shape, and also the symmetry factor ( g = / ) was determined. Using the symmetry factor, the order of kinetics is obtained from the Chens plot [52].
D.- Variable heating rate
During the TL readout of any order of kinetics, the heating rate is an important factor that affects the peak position of the TL glow curve. If the linear heating rate changes, the temperature corresponding to the maximum TL peak intensity also changes. Booth [53] suggested the use of two heating rates for the determination of trap depth as:
1
2
= 1 2
1 2
[2
2 2
2
(
2
)2] ..(12)
2
1
= {[ 2
1
1 ]} (13)
(1 2)
Where E is the trap depth, TM1 and TM2 are the peak temperatures corresponding to linear heating rates 1 and 2 and k is the Boltzmanns constant.
-
-
THERMOLUMINESCENCE PROPERTIES OF BORATES
Borate-based phosphors like lithium tetraborate (LTB) and magnesium tetraborate (MTB) are very close to tissue equivalent. Lithium tetraborates (LTB) are a very promising material due to their potential application and TL properties. The effective atomic number of lithium borate crystal is 7.3, which is very close to biological tissue of 7.42 and makes it attractive TLD materials for personal dosimetry [54]. Lithium tetraborate (Li2B4O7) is a congruently melting compound with a low melting point and small density (melting point = 916 °C, = 2.45 g/cm3) [55]. The crystal structure of LTB is identified by the space group I41cd and point group C4v. It consists of =BOB covalent network, which has a frame of alternating oxygen- bonded BO4 and BO3 oxyanions and is stabilized by Li+ ions accommodated within this network [56]. The basic structural unit of the LTB crystal is a cradle-like B4O7 group [57] and it exhibits tetragonal structure with lattice parameters a = b = 9.47 and c = 10.26 .
Fig. 3 – Structure of Li2B4O7 lattice shown as projections of part of the tetragonal elementary cell along a [110]-type axis.
There are various attractive features of LTB, such as high radiation resistivity, a wide operation dose range, and wide practical applications. The first TL dosimetry material based on borate was Lithium tetraborate doped with manganese [58]. This TL dosimetry material had a lesser sensitivity due to the incompatibility of red emission (600 nm) with the spectral response of the photomultiplier tubes. With the addition of copper as a dopant, the sensitivity of LTB materials increased by tenths times, and emission wavelength shifted from red region to ultraviolet region (360 nm) [59]. Additionally, the sensitivity of the TLD materials can also be increased by using dopants such as silver, phosphorus, magnesium, indium, or any combination of these dopants [60 62]. The first single crystals of LTB used for infrared transmission studies were produced by Garret et al. [63]. To study the structural properties and understand the mechanism of LTB phosphors, several research groups reported the synthesis of LTB single crystal. In 1980, the first time Polycrystalline powder samples of doped LTB phosphors were synthesized by the sintering method [59]. Later this technique was used [54, 60, 64] to develop LTB phosphors and studied their dosimetric characteristics.
Ozdemir et al [65] has studied the thermoluminescence dosimetric characteristics of Mn doped lithium tetraborateLTB powder and pellet samples which was synthesized by solution combustion technique. The starting materials lithium nitrate LiNO3 and boric acid H3BO3 were taken in stoichiometric proportion.
Fig. 4 – Schematic diagram of solution combustion synthesis of LTB:Mn
For the dosimetric properties of LTB: Mn material beta exposure was given at room temperature using 90Sr/90Y sources. The dose rate was 6.689 Gy/min. To study the TL curves powder and pellets of LTB: Mn exposed to a 2 Gy beta dose. This
curve exhibit two groups of TL maxima shown in fig. 5. The first TL peak is obtained at around 100 oc, is a low-temperature peak, and decays after exposure. The second TL peak is around 260 oc, which is an intense high-temperature peak used in the TL emission determinations. In the past years, various literature reported the same TL peaks obtained for the LTB [66 68].
Fig. 5 – TL glow curves for Li2B4O7:Mn powder and pellet samples exposed to 2 Gy beta dose.
Further analysis, using the TmTstop method, along with the IR method and CGCD technique, confirmed after the exposure of 5 Gy beta dose that the high-temperature glow peak could be separated into the TL peaks centered at 220, 268, and 292 °C with parameters as reported in Table1.
Table 1: Kinetic parameters estimated using IR and CGCD methods.
Activation Energy Ea (eV)
Kinetics Order (b)
Frequency Factor
(s) s-1
Methods
P1 22oC
1.03 ± 0.12
0.95 ± 0.01
–
1.46 ± 0.05
– 5.74 108
IR CGCD
P2 268oC
1.22 ± 0.05
1.22 ± 0.01
–
1.78 ± 0.07
– 2.32 1010
IR CGCD
P3 292oC
1.45 ± 0.04
1.44 ± 0.01
–
1.87 ± 0.05
– 5.65 1011
IR CGCD
The recycling and fading characteristics of studied phosphor have revealed important parameters such as linear dose-response at low doses, up to 10 Gy. The TL intensity increases linearly for both powder and pellets of LTB: Mn samples up to 10 Gy, and the dose-response curve followed superlinearity characteristics up to 100 Gy using beta rays exposure as depicted in fig. 6. After a 10 Gy beta dose exposure, the relative sensitivity of powder LTB: Mn samples is one-tenth of the sensitivity of TLD-
100. The LTB: Mn with different concentrations of Mn reported in the literature, which showed the same results for the dose- response studies [69]. Similar results were reported by Annalakshmi et al. for the dose values up to 10Gy for photon response of
0.32 wt% Mn-doped Li2B4O7 [67]. Danilkin et al. also studied TL dose dependencies for Mn-doped Li2B4O7, where two groups of samples showed supra-linearity nearly at doses from 1 to 500 Gy [70].
Fig. 6 – Dose response of powder and pellets LTB:Mn and TLD-chips exposed to beta rays
The calculated low photon energy dependence (less than 1MV), at 4080 keV and 662 keV, of LTB: Mn was higher than that of high energy photons to some extent. The fading effect of LTB: Mn was observed when stored fresh LTB: Mn powder samples were exposed to 1 Gy beta dose for 30 days in the dark and at room temperature with humidity around 46% RH in the laboratory.
At a low-temperature peak, strong fading was observed in 3 hours which completely faded within 12 hours. At a high intense temperature peak observed at 260 oc, the fading effect was 10% in one month. The maximum fading obtained in 7 days was 27%, but total fading is again 10% in one month. These LTB samples showed abnormal fading behavior with a small build-up in the initial part of the curve, on the other hand, normal decay was observed in the other literature for the various phosphors [71
74]
Fig. 7 – Effect of fading after different periods of storage in the dark and room temperature
It was observed that LTB: Mn 0.04M% phosphor is a promising material. Such a TLD material can be used for ionizing radiations, especially in clinical therapy dosimetry. Along with this, LTB compounds are commonly used as efficient materials for acoustic electronics, nonlinear optics, piezo-technology and different field of science and industry.
Kurali et al. adopted the czochralski method [75] to prepare the Ag-doped LTB crystals. The concentration of silver in LTB was 0.25 wt%. With the help of the Czochralski method, single crystals of LTB were grown and cut into a size of 5x3x1 mm, and then polished. The TL glow curve of Ag-doped LTB single crystal has four well-separated peaks located at 75, 130, 190,
and 275 oc, whereas undoped LTB has a broad peak at 125 oc and a sharp peak at 190 oc using a heating rate of 5 oc/s in the temperature range of 50 250 oc as shown in fig. 8. Petra et al. also studied the LTB: Ag single crystal using the czochraslki method in ambient air, but they reported only one intense peak at -155 oc at a temperature range of 50 250 oc [76]. This is due to the variation in the significant size of Li+ (76pm) and Ag+ (115pm) ions.
Fig. 8 – Comparison of TL glow curves of undoped (dashed line) and Agdoped (solid line) LTB single crystals, exposed to radiation (600 mGy) from a 90Sr 90Y source at room temperature.
It is observed from the dose-response of both Ag-doped and undoped single crystal, that the main peak of Ag: LTB at 275 oc has a linear response in the range of 33 800 mGy, and between 1 and 9 Gy it shows a sublinear region as shown in fig. 9. The undoped single crystal showed supralinear response for low doses and linear response in the range of 1 to 9 Gy observed from fig. 10.
Fig. 9 – TL Dose response of LTB:Ag single crystal
Fig. 10 – TL doseresponse of undoped LTB single
To test the thermal fading ratio of LTB: Ag and undoped single crystal, samples were irradiated with beta rays at 100 and 600 mGy, annealed, and then stored in the darkroom for seven days. It is observed that different behavior exhibited by Ag-doped LTB single over 7 days, whereas undoped LTB shows 60% fading for high-temperature peak after 7 days.
After the 10 repeated measurement cycles, TL responses of Agdoped and undoped single crystals did not show any considerable change under the same experimental conditions. The TL sensitivity of Li2B4O7: Ag was five times less than that of TLD100, which makes it suitable for radiation dosimetry, particularly in high-dose regions [75].
-
MAGNESIUM TETRABORATE PHOSPHORS(MBT)
Several researchers investigated that magnesium borates are used as TL material. In 1974 first time the preparation of polycrystalline magnesium borate doped with dysprosium has been reported by Kazankaya et al. [77]. This TL material becomes more important because of the near tissue equivalence (Zeff = 8.5) compared to that of tissue which is 7.4. After that, continuous efforts are being made to improve the dosimetric properties of magnesium borate-based phosphors. Dy/Tm doped magnesium tetraborate along with a sensitizer was developed in the year 1980 [78]. This material has a TL sensitivity which is almost seventh times that of TLD-100. With the addition of a co-activator, the spectral distribution of the phosphor has no influence but it only enhances the relative emission efficiency.
Magnesium tetraborate is suited for photon, beta, and neutron dosimetry. It reported that trace amounts of impurities like copper, nickel, iron, potassium, manganese, calcium, etc., cause a considerable reduction in the intensity of the principle dosimetric peak [79]. The dosimetric glow peak of Dy/Tm along with the sensitizers was nearly 200 °C. It is observed that the sensitivity of the phosphor is not the same in all the reports. Prokic studied the synthesis of manganese doped magnesium tetraborate [80]. Though this phosphor exhibited a dosimetric peak around 270 °C, the sensitivity of the phosphor changes depends on the observation taken by the instrument.
Therefore, the relative intensities of the maxima of the TL emission spectra change with the chemical form of the dopant. It is found that Tm doped magnesium tetraborate synthesized by sintering method at 900 °C, the dose-response is observed linear from 50 Gy to 6 kGy [81] and fading is 30 in 45 days. Due to the rapid use of ionizing radiation strictly quality control is required to avoid unnecessary irradiations to the healthy tissues in the medical field for both radiodiagnostic and therapeutic purposes. This has led to the utilization of tissue-equivalent or nearly tissue equivalent dosimeters for dosimetric applications, which has once again aroused the development of MgB4O7 based phosphors. MgB4O7 has excellent dosimetric properties, such as linear dose responses over broad absorbed dose range [82 83]; TL glow curves usually with a single peak; low fading, approximately 8% in three months for MgB4O7:Dy; and less than 5% in one month for MgB4O7:Ce, Li [83]. In addition to this, due to their high chemical stability (melting point above 1000 °C) and wide bandgap (9.5 eV), these matrices can produce a large variety of stable trapping and color centers [84]. Recently MgB4O7:Ce, Li has been referred to as an optically stimulated dosimeter (OSL) [83, 85-87].
Gonzalez et al. in his work described the effect of sintering temperature on the sensitivity of magnesium borate obtained by the solution combustion technique [88]. The stoichiometric amounts of magnesium nitrate, boic acid, and urea were mixed in a
poly-tetrafluoroethylene vessel, and TmCl26H2O and AgNO3 were added as an activator in the desired proportions. Tm
concentration varied from 0.125 to 1.5 mol %. Then to produce the combustion process, the mixture was perfectly dried and
placed in a pre-heated oven at 923 K [89 90]. Behind the combustion process to make dosimeter in the pellet of 0.8 mm thick and 0.5 mm in diameter, the obtained powders were pressed at 1 ton/cm2 using stainless steel molds. To compare the sensitivity
of the new prepared MgB4O7:Tm, Ag TL material, commercial dosimeters TLD100 chips, 3 × 3×1 mm3 [91], was used. Fig. 11 shows the glow curves of MgB4O7:Tm, Ag (0.5, 0.1 mol%) exposed to 1 Gy of 60Co gamma radiation of samples with heat treatment of sintering temperature at 1023, 1123, and 1223 K for 3hrs. The TL glow curves of samples sintered at 1023 and 1123 K, obtained the center peak at 497 K, while the sample sintered at 1223 K showed the center peak at 481 K.
Fig. 11 – Glow curves of MgB4O7:Tm,Ag (0.5, 0.1 mol%) sintered pellets at different temperature for 3 h, dose 1 Gy.
It was observed that when the Ag concentration was 0.1 mol% and Tm concentration is taken as 0.125 mol% sensitivity of the MgB4O7: Tm, Ag is 2.5 times higher than the sensitivity of the commercial dosimeter TLD-100 (LiF: Mg, Ti). As the Tm concentration increased to 0.25 mol%, the sensitivity of the MgB4O7:Tm, Ag reaches its maximum at 3.94 times higher than the sensitivity of the commercial dosimeter. Fig. 12 shows that linear TL response is obtained when the MgB4O7:Tm, Ag (0.5, 0.1 mol%) exposed to gamma radiation for the dose range 10 3-102 Gy. With the help of this method high-sensitivity tissue- equivalent, the material was established, which may be useful in clinical dosimetry applications [88].
Fig. 12 – TL response of MgB4O7:Tm,Ag as a function of 60Co gamma dose.
-
ZINC BORATE BASED PHOSPHORS (ZBT)
Zn-borate and its hydrates are extensively studied materials for different fields such as flame retardants, smoke suppressants, glow suppressants, corrosion inhibitors, and synergistic agents [92 94]. As compared to other rare earth borates, Zinc borate is an optical-thermal stable and cheaper compound. The crystal structure of the ZnB4O7 contains two BO3 triangles and two BO4
tetrahedra having a common vertex giving [B4O7]2 unit. There is a complete repetition of such units throughout the structure. Each zinc atom is enclosed by four neighboring oxygen atoms (ZnO4), arranged in an irregular and distorted tetrahedron manner.
In 2015, Luitel et al successfully synthesized a novel, ZnB4O7:Eu3+ phosphor by hydrothermal treatment. During hydrothermal treatment, nanorods were formed, have high-density contained urchin-like structures, and were radially arranged. The obtained nanorods were 200 to 400 nm in diameter and several m in length. The ZnB4O7:Eu3+ phosphor was efficiently excited by a 250450 nm light source, that showed intense orange-red emission consisting of dominant peaks at 590, 615, and 695 nm due to 5D07F1, 5D07F2, and 5D07F4 transitions of Eu3+ ions in the ZnB4O7 host. The optimum Eu3+ ions concentration for best PL intensity was observed to be at 5%. The -ZnB4O7 phase is stable only up to 798°C in an air ambient, and above that temperature, -ZnB2O4 phase transformation occurred. The temperature-dependent photoluminescence emission spectra of ZnB4O7:Eu shown in fig. 13.
Fig. 13 – Temperature dependent emission spectra of ZnB4O7:5at% Eu3+ phosphor. Inset is the comparison of ZnB4O7:5at% Eu3+ (em@611nm) with the commercial Y2O2S:Eu3+ phosphor (em@ 627nm).
The relative absorption cross-section experiment was done comparing with commercial Y2O2S: Eu3+ phosphor as observed in fig. 14.
Fig. 14 – PL absorption spectra of ZnB4O7:Eu phosphor compared with the Y2O2S:Eu phosphor
The results indicated that the ZnB4O7:Eu3+ is a promising phosphor with good thermal stability compared to the commercial Y2O2S: Eu3+ phosphor and has a potential application in UV pumped fluorescent lamps, as well as UV to blue, LED excited white display LEDs [95].
Bulcar et al. (2018) reported the dosimetric characteristics of Sm activated ZnB2O4 phosphors under beta irradiation and found that TL material exhibited the linear dose-response from 0.115 to 69 Gy when an optimal doping concentration of 2% Sm3+ is used [96]. Another borate-based TL material ZnB2O4:Tm prepared by a wet chemical method, in which TL glow curves consisted of three different peaks, located at 181 °C, 213 °C, and 351 °C and the Tm-Tstop investigations on regenerated TL signals revealed that there are five different traps with energy values in the range from 0.61 – 1.71 eV. Additionally, it is observed that for the beta-ray exposure the dose-response increased linearly for the three peaks in the dose range from 0.11 to 60 Gy. After exposure of 10 Gy dose, repeated for ten successive irradiation cycles to check reproducibility, and it was found that the maximum variation was less than 1% from the average value [97].
-
CONCLUSION
-
This review mainly focuses on borate-based materials and discusses their TL properties such as such as glow curve, dose response, annealing condition, TL sensitivity, fading and reproducibility. Boratebased materials play a crucial role in measuring the radiation dose of ionizing radiation and have a wide range of applications such as in radiation dosimetry, personal dosimetry, medical dosimetry, and in different areas of science and technology. Borate-based materials like lithium tetraborate and magnesium tetraborate are close to tissue equivalent. These materials are extensively beneficial for personal dosimetry. A lot of the research works have been done to improve the TL properties of borate-based materials, either by changing the preparation technique or by the use of different modifiers or activators. Thus, it is concluded that the sensitivity of borate-based TLD materials like LTB, MBT, and ZBT can be increased by using different dopants or co-dopants. In addition to this, due to their high chemical stability, wide bandgap, and great potential, these materials can produce a large variety of stable trapping and color centers. With the advancement and growing interest of TLD materials, especially in the fields of medical, environmental, and personal applications continuous efforts are being made to develop new TL D materials, having better tissue equivalence, and increased sensitivity to gamma radiation, high energy photons, electrons, neutrons, and heavy ions.
REFERENCES
[1] Deluca, J.A. (1980). Journal of Chemical Education, 57, 541 545 fig. (1). [2] Murthy, K. V. R., and Virk, H. S. (2014). Defect and Diffusion Forum, 347, 1 – 34. [3] Mckinlay, A.F. (1981). Medical Physics Handbook of Thermoluminescence Dosimetry. Adam Hilger Ltd, Bristol in collaboration with the Hospital Physicists Association. [4] Mckeever, S. (1985). Thermoluminescence of Solids. Department of Physics, Oklahoma state university, Cambridge University press. [5] Mckeever, S.W.S., and Chen, R. (1997). Theory of thermoluminescence and Related Phenomena. World scientific, Singapore. [6] Becker K. (1973). CRC Press, Inc., Cranwood Parkway, Cleveleand, Ohio 44128, U.S.A. [7] Wiedemann, E., and Schmidt, G.C. (1895). Über luminescenz. Ann. Physik., 54, 604-625. [8] E.Wiedemann, E. (1889). Zurmechanik des leuchtens. Ann. Phys-Berlin., 37, 177- 248. [9] Eduardo, G., Yukihara, Stephen, W. S., and McKeever, (2011). Optically Stimulated Luminescence: Fundamentals and Applications, John Wiley & Sons., UK. [10] Trowbridge, J., & Burbank, J.E. (1898). Am. J. Sci., Ser. 4, 5, 55. [11] Curie, M. (1961). Radioactive Substances, New York: Philosophical Library. [12] Bull, R.K. (1986). Thermoluminescence and its Applocations: An Introduction. Nucl. Tracts Radiat. Meas., 11, 105-113. [13] Wick, F.G., and Slattery, M.K. J. Opt. Soc. Amer 16, 398. [14] Wik, F.G., and Acad, S.B. (1938) Wiss Wien IIa, 139, 497 [15] Przibram, K. (1956). Irradiation Colours and Luminescence, (Pergamon Press London, London) [16] Randall, J.T., and Wilkins, M.H.F. (1945). Proc. Roy. Soc. A 184, 346. [17] Garlick, G.F.J., and Gibson, A.F. (1948). Proc. Phys. Soc. 60, 574. [18] May, C.E., and Patridge, J.A. (1964). J.Chem. Soc. 40, 1401. [19] Barthe J.R., Bohm, J., Christeasen, P., Driscoll, C.M., Harvey, J.R., Julius, H.W., Marshall, M., Marshall, T.O. and Oberhoffer, M. (1987). report on a Workshop on The Application of Thermoluminescence DosimetryTo Large Scale Individual Monitoring. Ispra, 11-13 september, 1985, Radiation Protection Dosimetry, Vol. 18. No.1. pp 47-61. [20] Li, J., Hao, J. Q., Li, C. Y., Zhang, C. X., Tang, Q., Zhang, Y. L., Su, Q. and Wang, S. B. (2005). Radiat.Meas. 39, 229. [21] Madhukumar, K., Varma, H. K., Komath, M., Elias, T. S., Padmanbhan, V. and Nair, C. M. K. (2007). Bull. Mater. Sci. 30, 527. [22] Pradhan, A. S. (1981). Radiation Protection Dosimetry, 1 (3), 153-167. [23] Ege, A. T., Ekdal, E., Karali, T., and Can, N. (2007). Radiation Measurements, 42, 1280-1284. [24] Sunta, C.M. (2015). Unraveling Thermoluminescence, Springer Series in Material Science, Springer Science + Business Media, [25] Rey, L., Gartia, R.K., Bishal K., Singh and Th. Basanta Singh, (2009). Nucl. Instr. and Meth. in Phys. Res. B, 267, 3633. [26] Nambi, K. S. V., Kathuria, S. P., and Sunta, C. M. (1969). Radiation Protection Monitoring, IAEA, Viena, 321. [27] Withnall, R., and Silver, J. (2012). Physics of light emission from rare-earth doped phosphors, Handbook of Visual Display Technology, 1019-1028, Springer-Verlag Berlin Heidelberg 2012 ed., Janglin Chen Industrial Technology Research Institute Taiwan Wayne Cranton Nottingham Trent University UK Mark Fihn Veritas et Visus USA. [28] Lakshmanan, A. (2016). The Role of Sintering in the Synthesis of Luminescence Phosphors, file:///C:/Users/ufs/Documents/Research%20data%202016/Books/Combustion% 20synthesis.pdf (accessed 20/6/2017). [29] Sawala, N. S., Palan, C. B., Chauhan A. O., and. Omanwar, S. K. (2016). Optik International Journal for Light and Electron Optics, 1 – 12, doi.org/10.1016/j.ijleo.2016.02.064. [30] Ingle, J.T., Sonekar, R.P., Omanwar, S.K.,Wang, Y., and Zhao, L. (2014). Combustion Science Technology, 186, 83 – 89. [31] Lephoto, M.A., Ntwaeaborwa, O.M., Pitale, S.S., Swart, H.C., Botha, J.R., Mothudi, B.M., Physica B. (2012). 407, 1603 – 1606. [32] Patil, K.C., and Mimani, T. (2001). Solution combustion synthesis of nanoscale oxides and their composites. Mater. Phys. Mech. 4. 134 137. [33] Huang, J., Zhou, L., Pang, Q., Gong, F., Suna J., and Wanga, W. (2009). Luminescence, 24, 363 – 366. [34] Li, P., Wang, Z., Yang, Z., Guo, Q., and Li, X. (2009). Materials Letters, 63, 751 – 753. [35] Zhang X., and Gong, M. (2014). Dalton Transactions, 43, 2465 – 2472. [36] Liu, W., Huang, C., Wu, C., Chiu, Y., Yeh. Y., and Chen, T. (2011). Journal of Material Chemistry, 216869 – 6874. [37] Wang, L., and Xie, R. (20170. Materials for Solid State Lighting and Displays, John Wiley and Sons Ltd, UK. [38] Anthony R. (2005). West Solid State Chemistry and its Applications", Wiley and Sons, [39] Gerand, B., Nowogrocki, G., Guenot, J., and Figlarz, M. "Preparative methods in Solid State Chemistry", Academic press [40] http://shodhganga.inflibnet.ac.in/bitstream/10603/40974/10/10_chapter%202.pdf (accessed on 20/06/2017). [41] Patnaik, P. (2004). Dean's Analytical Chemistry Handbook, 2nd ed. McGraw-Hill. [42] Harvey, D. (2000). Modern Analytical Chemistry. McGraw-Hill. [43] Shinde S.S., and Rajpure, K.Y. (2011). Fast response ultraviolet Ga-doped ZnO based photoconductive detector, Materials Research Bulletin, vol. 46, no. 10, pp. 17341737. View at: Publisher Site Google Scholar. [44] Ullah R., and Dutta, J. (2008). Photocatalytic degradation of organic dyes with manganese-doped ZnO nanoparticles, Journal of Hazardous Materials, vol. 156, no. 1-3, pp. 194200. View at:Publisher Site | Google Scholar [45] Hossain, M.K., Ghosh,S.C, Boontongkong, Y., Thanachayanont, C., and Dutta, J. (2005). Growth of zinc oxide nanowires and nanobelts for gas sensing applications, Journal of Metastable and Nanocrystalline Materials, vol. 23, pp. 2730. View at: Publisher Site | Google Scholar. [46] Natsume Y., and Sakata, H. (2000). Zinc oxide films prepared by sol-gel spin-coating, Thin Solid Films, vol. 372, no. 1-2, pp. 3036. View at: Publisher Site | Google Scholar. [47] Kitis G., Furetta C., Prokic M., amd Prokic V. (2000). J. Phys D: Appl Phys. 33, 1252. [48] Randall J.T., and Wilkins M.H.F. (1945). Proc. Roy. Soc. London. 184, 365. [49] Randall J.T., and Wilkins M.H.F. (1945). Proc. Roy. Soc. London. 184, 390. [50] Bos. A.J.J. (2001) Nucl. Instr. Methods in Phys. Res. B. 184, 3. [51] Rasheedy M.S. (1993). J. Phys.: Condens. Matter 5, 633. [52] Chen R., and Winer S.A.A. (1970). J. Appl. Phys. 41, 5227. [53] Booth A.H., and Can J. (1954). J. Chem. Ottwa. 32, 214. [54] Furetta C., Prokic M., Salamon R., Prokic V., and Kitis G. (2001). Dosimetric characteristics of tissue equivalent thermoluminescent solid TL detectors based on lithium borate,Nuclear Instruments and Methods in Physics Research, SectionA: Accelerators, Spectrometers, Detectors and Associated Equipment, vol. 456, no. 3, pp. 411417. [55] Ishii M., Kuwano, Y., Asaba, S., Asai, T., Kawamura, M., Senguttuvan, N., Hayashi, T., Koboyashi, M., Nikl, M., Hosoya, S., Sakai, K., Adachi, T., Oku, T., Shimizu, H.M. (2004). Radiation Measurements 38, 571 574. [56] Krogh-Moe, J. (1962). Acta Crystallogr. 15, 190. [57] Corradi, G., Nagirnyi, V., Kotlov, A., Watterich, A., Kirm, M., Polgár, K., Hofstaetter A., Meyer, M. (2008). J. Phys.: Condens. Matter 20, 025216 (9pp). [58] Schulman, J. H., Kirk, R. D., and West, E. J. (1967). Proceedings of the International Conference on Luminescence Dosimetery, Stanford University.CONF-650637, 113.
[59] Takenaga, M., Yamamoto, O., and Yamashita, T. (1980). Preparation and characteristics of Li2B4O7: Cu phosphor. Nucl. Instr. and Meth. 175, 77 78. [60] Huy, B.T., Quang, V.X., and Chau, H.T.B. (2008). Effect of doping on the luminescence properties of Li2B4O7. J. Lumin. 128, 1601 – 1605. [61] Lakshmanan, A.R., Chandra, B., and Bhatt, R.C. (1981). Dosimetry characteristics of thermoluminescent Li2B4O7:Cu phosphor. Radiat. Prot.Dosim. 1 (3), 191 – 198.
[62] Prokic, M. (2001). Lithium borate solid TL detectrors. Radiat. Meas. 33, 393 – 396. [63] Garrett J.D., Natrajan-Iyere M., and Greedan, J. (1977). Cryst. Growth, 41, 225. [64] El-Faramawy N.A., El-Kameesy S.U., El-Aframy A., and Metwally G. (2000). Radiat.Phys. Chem., 58, 9. [65] Ozdemir A., Yegingil Z., Nur N., Kurt K., Tuken T., Depci T., Tansug G., Altunal V., Guckan V., Sigircik G., Yu Y., Karatasli M., and Dolek Y. (2016). Thermoluminescence study of Mn doped lithium tetraborate powder and pellet samples synthesized by solution combustion synthesis, Journal of Luminescence 173, 149-158. [66] Manam J., and Sharma S. K. (2008). Determination of trapping parameters from thermally stimulated luminescence glow curves of Mn-doped Li2B4O7 phosphor, Radiat. Eff. Defects Solids 163 (10), 813 819. [67] Annalakshmi O., Jose M.T., and Amarendra G. (2011). Dosimetric characteristics of manganese doped lithium tetraborate An improved TL phosphor. Radiat. Meas. 46, 669- 675. [68] Annalakshmi O., Jose M.T., Madhusoodanan U., Venkatraman B., and Amarendra G. (2013). Kinetic parameters of lithium tetraborate based TL materials, J. Lumin. 141, 6066. [69] Langmead W.A., Wall B.F. (1976). TLD, System based on lithium borate for measurement of doses to patient undergoing medical irradiation,Phys. Med. Biol. 21 (1), 3951.
[70] Danilkin, M., Jaek, I., Kerikmäe, M., Lust, A., Mändar, H., Pung, L, Ratas, A., Seeman,V., Klimonsky, S., Kuznetsov, V., (2010). Storage mechanism and OSL-readout possibility of Li2B4O7:Mn (TLD-800), Radiat.Meas.45(36), 562565. [71] Spurny Z., and Kvasnika J. (1974). Short-term fading of different thermoluminescent phosphors, in: Proceedings of the Fourth International Conference on Luminescence Dosimetry, Institute of Nuclear Physics Krakow, Poland, 1, pp. 255262. [72] Nambi K.S.V. (1977). Thermoluminescence: its understanding and applications, Atomic Energy Agency Publications, Sao Paulo, Brasil. [73] Furetta C., and Pellegrini R. (1981). Some dosimetric properties of Li2O7:Mn (TLD-800), Radiat. Eff. Defects Solids, 58, 1723. [74] Horowitz Y.S. (1984). Thermoluminescence and thermoluminescent dosimetry, CRC Press, Boca Raton, FL. [75] Kural D., Ekdal K.E., Kelemen A., Holovey V., Can N. and Karal T. (2016). Thermoluminescence characterization of Agdoped Li2B4O7 single crystal materials. journal of biological and chemical luminescence, 32, Pp 786790. [76] Patra G.D., Singh S.G., Singh A.K., Tyagi M., Desai D.G., Tiwari B., Sen S., and Gadkari S.C. (2015). Silver doped lithium tetraborate (Li2B4O7) single crystals as efficient dosimeter material with sub-micro-Gy sensitivity. J. Lumin. 157, 333. [77] Kazankaya V. A., Kuzmin V.V., Minaeva E.E., and Sokolov A.D. (1974). Proc. 4th Int.Conf. Luminescence Dosimetry, Kracow, Poland, 581. [78] Prokic M. (1980). Development of highly sensitive CaSO4: Dy/Tm and MgB4O7: Dy/Tm sintered thermoluminescent dosimeters. Nucl. Instrum.Methods, 175, 83.
[79] Prokic M. (1986). Magnesium Borate in TL Dosimetry. Radiat. Prot. Dosim. 17, 393-396. [80] Prokic M. (1993). MgB4O7:Mn as a New TL Dosemeter. Radiat. Prot. Dosim., 47, 191-193. [81] Porwal N.K., Kadam R.M., Seshagiri T.K., Natarajan V., Dhohale A.R., and Page A.G., (2005). Radiat. Meas. 40, 69. [82] Pekpak, E., Ylmaz, A., and Özbayoglu, G. (2010). An overview on preparation and TL characterization of lithium borates for dosimetric use. Int. J. Miner. Process. 3, 1424. [83] Souza, L.F., Silva, A.M.B., Antonio, P.L., Caldas, L.V.E., Souza, S.O., d'Errico, F., and Souza, D.N. (2017). Dosimetric properties of MgB4O7:Dy,Li and MgB4O7:Ce,Li for Optically stimulated luminescence applications. Radiat. Meas. 106, 196199. [84] Oliveira, T.M., Lima, A.F., Brik, M.G., Souza, S.O., and Lalic, M.V. (2016). Electronic Structure and Optical properties of magnesium tetraborate:an ab initio study. Comput. Mater. Sci. 124, 17.
[85] Yukihara, E.G., Milliken, E.D., Oliveira, L.C., Orante-Barro, V.R., Jacobsohn, L.G., and Blair, M.W. (2013). Systematic development of new thermoluminescence and optically Stimulated luminescence materials. J. Lumin. 133, 203210. [86] Yukihara, E., Milliken, E., and Doull, B. (2014). Thermally stimulated and recombination processes in MgB4O7 investigated by lanthanide doping.J. Lumin. 154, 251259.
[87] Yukihara, E.G., Doull, B.A., Gustafson, T., Oliveira, L.C., Kurt, K., and Milliken, E.D. (2017). Optically stimulated luminescence of MgB4O7:Ce,Li for gamma and neutron dosimetry. J. Lumin. 183, 525532. [88] Gonz´alez, P.R., Avila, O., Mendoza-Anaya, D., and Escobar-AlarconL. (2021). Effect of sintering temperature on sensitivity of MgB4O7:Tm,Ag obtained by the solution combustion method, Applied Radiation and Isotopes, 167, 109459. [89] Kingsley, J.J., Suresh, K., and Patil, K.C. (1990). Synthesis of fine-particle metal aluminates. J. Mater. Sci. 25, 13051312. [90] Gonzalez, P.R., Mendoza-Anaya, D., Virafuentes-Ch´avez, H.J., and AzorÃn, J. (2018). Dosimetric properties of -Al2O3:Tm+PTFE phosphor. Appl.Radiat. Isot. 141, 162166.
[91] Thermo Scientific. TLD materials specifications: features and technical specifications. https://assets.thermofisher. com /TFS Assets/LSG/Catalogs/ Dosimetry-Materials-Broch ure.pdf. (Accessed 2 March 2018). [92] Ting, C., Jian-Cheng, D., Long-Shuo, W., Fan, Y., and Gang, F. (2008). Synthesis of a new netlike nano Zinc borate, Materials Letters, vol. 62, no. 14, pp. 20572059. View at: Publisher Site | Google Scholar. [93] Samyn, F., Bourbigot, S., Duquesne, S., and Delobel, R. (2007). Effect of zinc borate on the thermal degradation of ammonium polyphosphate,Thermochimica Acta, vol. 456, no. 2, pp. 134144. View at: Publisher Site | Google Scholar.
[94] Genovese A., and Shanks, R.A. (2007). Structural and thermal interpretation of the synergy and interactions between the fire retardants magnesium hydroxide and zinc borate, Polymer Degradation and Stability, vol. 92, no. 1, pp. 213. View at: Publisher Site | Google Scholar. [95] Luitel H M, Chand R., and Watar T. (2015). A Novel Orange-Red Emitting ZnB4O7:Eu3+ Phosphor with Urchin-Like Nanostructure, Advances in Condensed Matter Physics https://doi.org/10.1155/2015/797056. [96] Bulcar, K., Dogan, T., Akça, S., Yüksel, M., Ayvacikli, M., Karabulut, Y., Kucuk, N., Canimoglu, A., Can, N., and Topaksu, M. (2018). Thermoluminescence behavior of Sm3+ activated ZnB2O4 phosphors synthesized using low temperature chemical synthesis method. Nucl. Instrum. Methods. Phys. Res. B: Beam Interactions with Materials and Atoms 428, 6571. [97] Bulcar, K., Kucuk, N., Topaksu, M., and Can, N. (2019). Thermoluminescence spectra of Tm doped ZnB2O4 phosphor prepared via a wet-chemical synthesis. Appl. Radiat. Isot.147, 177181.