
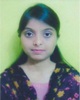
- Open Access
- Authors : Huma Yasmin , Nirjhar Dasgupta
- Paper ID : IJERTV12IS040237
- Volume & Issue : Volume 12, Issue 04 (April 2023)
- Published (First Online): 30-04-2023
- ISSN (Online) : 2278-0181
- Publisher Name : IJERT
- License:
This work is licensed under a Creative Commons Attribution 4.0 International License
Plant Responses to Sustain Abiotic Stress – A Review
Huma Yasmin1, Nirjhar Dasgupta2*
1 Department of Life Science, Guru Nanak Institute of Pharmaceutical Science and Technology, 157/f, Nilgunj Rd, Panihati, Kolkata, West Bengal; 2 Biotechnology Department, Techno India University,
EM-4/1, Sector-V, Salt Lake, Kolkata-700091
Abstract:- Drought, cold, excessive salt, and heat are key abiotic factors that limit food crop yields globally. Due to the multigenic nature of stress tolerance, traditional plant breeding techniques to improve agricultural abiotic stress tolerance have had little effectiveness. Molecular approaches have been employed in the last decade to study the mechanisms by which plants detect environmental cues and transmit them to cellular machinery to trigger adaptive responses. This understanding is crucial for the development of rational breeding and transgenic techniques to improve agricultural stress tolerance. Several genes involved with stress adaptation have been identified as a result of research into the physiological and molecular processes of abiotic stress tolerance. Microarrays, for example, have shown to be quite useful in establishing a list of stress-related genes. Some of these genes are exclusive to single stress, while others are shared by several stressors. Surprisingly, many genes are involved in both abiotic and biotic stress responses. This demonstrates the complexities of plant stress response and adaptability. Abiotic stress tolerance involves a whole gene cascade, beginning with stress perception and progressing to transcriptional activation of downstream genes, which leads to stress adaption and tolerance. A lot of these genes have been identified, but we do not yet have a full list of all interactions. A considerable number of genes with unknown functions have also been discovered to be controlled by abiotic stressors. Understanding the function of these genes and their interactions with other known genes to influence stress adaption is necessary. The new finding that microRNAs affect gene expression complicates our knowledge of abiotic stress tolerance. A substantial amount of study will be required to discover microRNAs related to abiotic stress response, as well as to comprehend their interaction with one another and the mechanism by which they regulate the abiotic stress response. The advancement of next-generation sequencing technology has enabled deep sequencing of mRNAs and microRNAs related to the abiotic stress response. In the near future, a thorough knowledge of physiological and molecular processes, particularly signalling cascades in response to abiotic stressors in tolerant plants, will aid in manipulating vulnerable crop plants and increasing agricultural production.
Keywords: Abiotic Stress, Catalase, ROS, H2O2, Superoxide, Homeostasis
INTRODUCTION:
The detrimental influence of non-living forces on living organisms in a certain habitat is referred to as abiotic stress [1]. The non- living variable must influence the environment beyond its typical range of fluctuation to have a significant negative impact on population performance or the individual physiology of the creature [2]. The survival, biomass production, and yields of staple food crops are significantly impacted by the primary abiotic stresses (drought, high salinity, cold, and heat) by up to 70%, endangering global food security [3]. The most common abiotic stress that inhibits plant growth and productivity is dehydration stress, which is brought primarily by drought, salinity, and temperature severity [4]. Understanding the main molecular pathways for advanced selective breeding is extremely difficult since resistance to this stress is multigenic and quantitative in nature [5]. The tolerances to these pressures have only slightly improved using conventional plant breeding methods. To better understand how stress signals are perceived and transmitted by the related molecular regulatory network, several high throughput sequencing and functional genomics approaches are being used to elucidate the molecular processes underpinning abiotic stress tolerance in plants [6]. For the creation of logical breeding and transgenic techniques to confer stress tolerance in crops, it is essential to comprehend the processes by which plants receive environmental signals and further their transmission to cellular machinery to trigger adaptive responses [7]. In the end, combining knowledge of the physiological, biochemical, and gene regulatory networks will be crucial to design or choosing cultivars of stress-tolerant, high-yielding food crops. The most important factors limiting plant geographical ranges on Earth are abiotic pressures such as high or low temperature, drought, and salt. Abiotic stresses typically result in significant crop yield losses. Abiotic stressors have an impact on the worldwide agricultural production system and jeopardize human food security.
Abiotic variables are responsible for 50% of crop output losses, according to global estimates [8]. The majority of them include high temperature (40%), salinity (20%), drought (17%), low temperature (15%), and various types of stress [9]. Only 9% of the world's land surface is suitable for agricultural production, while the remaining 91% is subject to numerous stressors. According to current estimates, 120.8 million people in India are degraded owing to soil erosion, salinity/alkalinity, soil acidity, waterlogging, and other edaphic concerns. Further significant productivity loss owing to rising detrimental impacts of abiotic stressors might be envisaged in the context of global warming and climate change [10].
Chilling damage in plants is caused by the individual components' susceptibility to low temperatures. The essential component that directly links plants that are vulnerable to chilling stress is cellular membrane integrity [11]. Plants that have more fatty acids in their cell membranes may endure greater chilling or frost stress, thus there must be some changes in the classes of these unsaturated fatty acids for plants to tolerate frost or chilling stress [12]. Under extremely low temperatures, different enzyme levels and activity rise or decrease. Several changes in physicochemical states occur in plant cell membranes, which compensate
for the effect of cold or frost by increasing cell membrane permeability and also create ionic and pH imbalance, eventually lowering ATP [13].
The ability of plants to withstand cold temperatures varies greatly. Plants cultivated in tropical and subtropical climates (for example, maize, cotton, soybean, rice, mango, tomato, and so on) are more susceptible to freezing. Plants growing in temperate climates may withstand low temperatures, however, the degree of tolerance varies by species. Furthermore, the great freezing resistance of these plants is not innate, as plants activate multiple physiological and biochemical systems to cope with freezing at low temperatures, a process known as 'cold acclimation.' In one research, rye plants exposed to -5 C without prior cold acclimatization could not survive, but when cold acclimatized at 2oC for 7-14 days, they were able to survive temperatures as low as -30 oC [14].
Plants exposed to extreme temperatures experience a variety of different alterations and responses. Adaptation to high temperatures occurs at various time frames and plant organization levels. Long-term exposure of plants to excessively high temperatures can result in serious harm and death. Different plant sections are impacted differently at such temperatures. The type of injury is determined by the plant's development stage, vulnerability, and cellular activities occurring at the moment. Heat, on the other hand, not only harms the plant at the cellular level but also impacts numerous complex processes and structures, finally leading to the plant's death [15].
Salt stress is a serious issue in arid and semiarid locations globally, affecting agricultural productivity and crop yields. Salinity affects around 20% of agricultural and 50% of irrigated land, reducing crop output and productivity [16]. Soil salinity can affect seed germination by raising osmotic potential outside the seeds, preventing water intake, or by the toxic effects of Na+ and Cl- ions on developing seeds [17].
Living beings are distinguished by two characteristics: cellular structure and a need for water. Even though the cellular origins of life might be debated, particularly in light of evolutionary scientific advances, the need for water reigns supreme. If free water is available, life forms can utilize any biological specialty, no matter how exceptional. Water is essential to plants because it performs several key processes. Herbaceous plants hold 90% of their fresh weight in water, according to Kramer and Boyer (1995). Water supports the turgor of plant cells, enabling respiration. Water also has various biophysical properties that make it a useful solvent (for example, high-temperature vaporization and enhanced surface tension). Drought accounts for over half of worldwide crop loss. Almost all farmed regions throughout the world are experiencing water scarcity. Drought stress or water scarcity is largely unpredictable flooding, however, 'dry seasons' are foreseen in some locations.
Land flooding is caused mostly by over-irrigation, inadequate drainage, and high rainfall [18]. Waterlogging is today a major problem, not just in locations where rainfall occurs, but also in areas where irrigation water is used. Flooding affects around 0.7 million acres in certain nations, and 60 thousand acres are always flooded due to inadequate drainage and water leakage through water channels.
Metal transfer into roots is accelerated in corrosive soils. In greater quantities, heavy metals (iron, manganese, copper, and zinc) can harm plant growth by affecting root development, reducing photosynthesis, and inhibiting various enzymes, which can lead to cell damage. However, many plant species have developed genetic and physiological tolerance to thrive in ordinary metal-rich soils or soils contaminated with higher levels of heavy metals [19].
MECHANISMS OF PHYSIOLOGICAL AND MOLECULAR ABIOTIC STRESS TOLERANCE:
-
HOMEOSTASIS AND ION TRANSPORTATION:
According to one theory, the effect of salinity on plant growth restriction results from an osmotic effect from the ion imbalance in the first phase and a direct influence of the ions themselves in the latter phase of mild to moderate stress [20]. Salt-sensitive species are unable to regulate Na+ transport at high salinities when ionic effects predominate over the osmotic impact. Plant cells require high K+ (100200 mM) and low Na+ (less than 1020 mM) levels to sustain proper metabolic processes [21]. Therefore, maintaining or fast re-establishing both osmotic and ionic homeostasis is required for tolerance to salt stress [22].
To maintain internal osmotic and ionic equilibrium to survive in highly salinity settings, plants often use one or both of the following strategies:
-
Avoidance: Keep delicate plant tissues away from areas where salt ions are concentrated.
-
Tolerance: to compartmentalize ions out of the cytoplasm of physiologically active cells, or to exclude ions from roots [23].
The primary adaptive tolerance responses to salt stress are, in fact, the effective exclusion of excess Na+ ions from the cytoplasm and the build-up of Na+ ions within vacuoles. Na+ is normally excluded from the cytosol in exchange for H+ by transmembrane transport proteins [24]. This secondary transport pathway is energy-dependent and propelled by the proton motive force produced by the plasma membrane H+ ATPase. Likewise, vacuolar membrane H+ ATPase and H+ pyrophosphatase proteins often carry out compartmentalization [25]. Numerous abiotic stress- tolerant transgenic plants have been created to regulate transport activities by boosting the cellular amounts of proteins (such as vacuolar antiporter proteins). It has been discovered that the Arabidopsis gene At SOS encodes a plasma membrane Na+/H+ antiporter (NHX) with a considerable resemblance in sequence to the corresponding antiporter from bacteria and fungi [26]. Arabidopsis thaliana vacuolar H+ translocating pyrophosphatase (AVP1) or the vacuolar Na+/H+ antiporter (NHX1) gene overexpression accelerated the pumping of Na+ into the vacuole and
raised both accumulation and tolerance to Na+ [27]. By lowering the cytosolic Na+ concentrations, more effective sequestration of these ions to the vacuole may increase tissue tolerance to salt. Transgenic plants overexpressing AtNHX1 have further proven the significance of Na+ sequestration in salt tolerance [28].
-
-
OSMOTIC MODIFICATIONS AND REGULATING VARIABLES:
Cellular dehydration results from intracellular water loss brought on by salt, dryness, and cold. Plants store a wide range of organic substances, including a variety of sugars (mostly fructose and sucrose), sugar, alcohols, complex sugars (such trehalose and fructans), organic acids, and amino acids (proline), to avoid this and safeguard the cellular proteins (oxalate, malate) [29]. These metabolites can build up to high concentrations without disrupting intracellular biochemistry and are also known as compatible solutes or osmoprotectants [30]. The prevention of water loss and facilitation of osmotic adjustment is achieved by lowering the water potential inside the cell [31].
Through modifying enzymes that manufacture particular osmolytes, transgenic research has been conducted to create tolerant genotypes [32]. Since the role of the targeted osmolytes is not limited to osmotic adjustment but also confers osmoprotectant properties, the efficacy of this research on enhancing stress tolerance has varied [33]. Studies have shown that osmolyte buildup offered protection by scavenging reactive oxygen species (ROS) and performing chaperone-like tasks to maintain protein structures and functions [34]. Due to disruptions in endogenous pathways of basic metabolisms, pleiotropic consequences such as necrosis and growth retardation were also seen. In contrast to PEG-stressed plants, which depended on sugar build-up, salt-stressed plants appeared to utilize salt as an osmoticum, according to research by Patade et al. (in press).
Many transgenic research have been conducted to overexpress the genes for osmoprotectants like glycine-betaine [35] and proline [36]. Additionally, the engineering of compatible-solute overproduction has been focused on a variety of sugars and sugar alcohols (mannitol, trehalose, Myo-inositol, and sorbitol), safeguarding the membrane and protein complexes under stress [37]. An increasing corpus of research has focused in particular on the metabolism of trehalose as a way of developing stress resistance in plants [38]. The yeast trehalose-6-phosphate synthase gene was used to increase trehalose synthesis in transgenic tomatoes, which significantly increased their tolerance to salt, drought, and oxidative stress [39]. Similar to this, transgenic plants bred to overexpress polyamines demonstrated enhanced tolerance to a variety of abiotic stressors, including heavy metals, salt, drought, low and high temperature, and fungal disease resistance [40]. Along with osmotolerance, osmolyte accumulation is essential for preserving cellular functions. For instance, increased osmotolerance was achieved by overexpressing the P5CS gene in Medicago truncatula, and it also helped to preserve nitrogen-fixing activity under osmotic stress [41]. Engineering mechanisms for the overproduction of suitable solutes should be through stress-inducibl and/or tissue-specific regulation to reduce potential negative pleiotropic consequences as those outlined before [42].
-
COLD ACCLIMATIZATION:
Plants can withstand freezing temperatures or avoid them altogether, mostly by supercooling the water in their tissues. A process known as cold acclimation allows some plant species to enhance their freezing tolerance (FT) in response to low non-freezing temperatures (below 10°C) during a brief photoperiod [43]. When returning to a warm, non-acclimating climate, the degree of FT gained by cold acclimation is quickly lost since it is not constant and might change periodically. Osmotic stressors [44] and abscisic acid [45] therapy can both cause FT. Overwintering tissues exhibit a tendency to dehydrate deliberately, which at least partially causes FT [46]. Furthermore, once development stops in response to exposure to low temperatures, altered source-sink connections cause cellular alterations that lead to the build-up of storage proteins, carbohydrates, and starch [47]. The failure of an Arabidopsis sucrose synthase mutant to adapt to the cold served as evidence that sugar build-up is necessary for cold acclimation [48]. A role in osmoregulation is suggested by a large number of sugars in cold-acclimated plants, whereas less abundant sugars may function as signaling molecules or in cryoprotection [49].
Clarifying the physiological and molecular factors behind freezing tolerance has recently advanced. A vast number of genes are thought to be impacted by low temperatures, making up to 25% of the transcriptome, and this is mirrored in FT, a genetically complicated characteristic [50]. During the process of acclimating to cold temperatures, the expression of certain c cold-responsive -COR genes cause several physiological and biochemical changes, and the overall effect of the gene products is reflected in the level of FT achieved [51]. Several signaling pathways are responsible for controlling the activation of the COR genes when the LT stimulus is present [52]. The regulatory route involved in acclimating to cold is most likely the CBF (C-repeat binding factor) cold response pathway seen in A. thaliana. This is accomplished by quickly inducing CBF transcription factors in the presence of cold, which is followed by the production of the regulon genes, which increase freezing resistance [53]. Three CBF genes, CBF1 (DREB1b), CBF2 (DREB1c), and CBF3 (DREB1a), were specifically activated in Arabidopsis within 15 min of low-temperature exposure [54]. These CBFs represent members of the AP2/ERF (Apetala2/Ethylene response element binding factor) family of transcription factors [55], which are closely linked to one another [56]. It interacts with the dehydration-responsive element (C-repeat) The promoters of genes targeted by CBF contain DNA regulatory elements [57]. Many CBF regulon genes are activated by the CBF proteins [58]. As a result, cold tolerance rises [59]. By building up low-molecular-weight cryoprotective
metabolites such as raffinose, sucrose, and proline. In addition to the creation of cryoprotective polypeptides like COR15a [60].
In the early phases of cold acclimation, membrane rigidification and Ca2+ inflow are connected via cytoskeletal remodeling, which is necessary for the formation of maximal FT [61]. Changes in cytosolic calcium brought on by low temperatures are correlated with the expression of cold-responsive genes and the onset of FT. The fast cold-induced release of calcium from both extracellular and vacuolar reserves is what causes Arabidopsis to experience a rise in cytosolic calcium levels [62]. Active Ca2+ transporters help cells' Ca2+ homeostasis return to resting levels after the cold stimulation. The induction of DREB genes is associated with calcium spikes and cold-regulated gene expression [63]. CBF1, a DREB1A homolog, was overexpressed, which improved freezing-stress resistance and boosted the expression of cold-regulated genes (cor15a, cor6.6, and cor47) [64]. The cross-stress protective role of this gene family is demonstrated by the increased drought and salt tolerance that resulted from DREB1A overexpression in transgenic plants [65].
A fast influx of calcium into the cytosol is necessary for the typical cold activation of the CBF target genes KIN1 and KIN2 in Arabidopsis [66]. Dehydrin accumulation, a protein that builds up in vegetative tissues during dehydration stress, has been associated with the emergence of FT in both herbaceous and woody plants [67]. Recently, it was discovered that wheat (Triticum aestivum) vernalization completion and the up-regulation of low temperature-associated proteins are closely related [68].
-
ANTIOXIDANT PROTECTION FOR ABIOTIC STRESS TOLERANCE:
Aerobic activities regularly create reactive oxygen species (ROS) in chloroplasts, mitochondria, and peroxisomes, including singlet oxygen, hydrogen peroxide molecules, superoxide, and hydroxyl radicals [69]. The elevated levels of ROS are frequently linked to exposure to biotic (such as pathogens or pests) and abiotic (such as high light, UV radiation, temperature extremes, heavy metals, air pollutants, drought stress, salt stress, mechanical/physical stress) factors [70]. ROS are thought to be crucial to downstream defense/tolerance responses. Overproduction of ROS causes oxidative damage, including cell death [71] and lipid peroxidation of membranes [72]. Plants have enzymes and non-enzymatic metabolites that may be crucial in controlling ROS levels and preventing oxidative damage to cells [73].
In plant cells, lipid-soluble antioxidants like a-tocopherol and carotenoids, water-soluble reductants like glutathione and ascorbate, and antioxidant enzymes like catalase, ascorbate peroxidase, and superoxide dismutase (SOD, EC 1.15.1.1) work to counteract the negative effects of ROS [74]. In reaction to stress, several osmolytes build up in plant cells in addition to their function in neutralizing free radicals and safeguarding enzymes [75]. Tolerating oxidative stress requires the capacity to activate protective mechanisms, such as an increase in the activity of scavenging enzymes. By overexpressing the enzymes involved in oxidative protection, detoxification techniques have been used in transgenic enhancements for abiotic stress tolerances. For instance, overexpression of tobacco glutathione-S-transferase (GST) and glutathione peroxidase (GPX) decreased oxidative damage in the stressed transgenic seedlings while heat or salt stress treatment impaired the development of wild tobacco and enhanced lipid peroxidation [76]. Ascorbate peroxidase (APX) and CuZn superoxide dismutase (SOD) overexpression also improved drought stress tolerance and recovery in transgenic sweet potatoes. This resulted from a considerable rise in the expression of antioxidant enzymes, which also resulted in a decrease in electrolyte leakage and malondialdehyde levels [77]. Antisense barley 2-cysteine peroxiredoxin sequence also led to increased expression of APX and monodehydroascorbate reductase in Arabidopsis [78].
In transgenic Arabidopsis exposed to cold, overexpression of an alternate oxidase (AOX) gene decreased oxidative damage [79]. Since Vitamin-E deficient Arabidopsis mutants were chilling sensitive, it was shown that Vitamin E was another player in the defense system against oxidative stress. It was suggested that this was caused by an improper export of photoassimilate [80].
-
ABIOTIC STRESS-RELATED SIGNAL TRANSDUCTION: SPECIFICITY AND CROSS-TALK:
Abiotic stressors are multi-sensory complex stimuli (such as ionic imbalance and osmotic stress) that change the expression of several genes. The chain of molecular reactions starts with the detection of stress and progresses through signal transduction to the cytoplasm and nucleus, gene expression, and eventually metabolic adjustments that result in stress tolerance. Calmodulins, calmodulin-like proteins, calcineurin B-like proteins, and calcium-dependent protein kinases are the four main families of calcium-binding proteins that regulate the fast rise in cytosolic Ca2+ levels in response to the diverse environmental stress stimuli (CDPKs) [81]. Signals after the Ca2+ influx are suggested to be mediated by members of the Ca2+ dependent protein kinase (CDPK) gene family and are hypothesized to regulate combinations of phosphorylation/dephosphorylation cascades [82]. There is evidence that members of the CDPK family can stimulate ABA/stress-responsive gene expression. Tolerance to cold, salt, and drought stress has been linked to altered Oryza sativa CDPK (OsCDPK) expression [83].
Plants exhibit both stress-specific and common responses that shield them from various environmental stresses [84]. Plants regulate gene expression in response to stress, which results in both common and unique changes in the transcript levels of genes that respond to stress [85]. It has been noted that the gene expression caused by various pressures overlaps [86]. Under conditions of salt, drought, and cold stress, plants everywhere seem to experience osmotic and oxidative
stress [87]. However, osmotic stress is prevented by stress-specific and general tolerance mechanisms. For instance, ice nuclei production must be avoided or stopped to sustain endurance during freezing-induced osmotic stress [88].
In contrast, osmotic adjustment preserves osmotic homeostasis in the case of salt stress. Two classes of transcription factors are controlled by either abiotic stress alone (class I) or both biotic and abiotic stimuli together (class II) in Arabidopsis. Abiotic stressors such as salinity, osmotic, cold, and jasmonic acid treatments had a preference for inducing about 20 genes in the class I group. Athb-8 [89], CCA1, and DRE/CRT binding factors induced by cold stress are some of these transcription factors. Myb proteins, bZIP/HD-ZIPs, and proteins with the AP2/EREBP domain [90]. Additionally, Seki et al. (2002) used a full-length cDNA microarray that contained 7,000 distinct Arabidopsis cDNAs to find target genes that are induced by cold, drought, and salinity as well as members of the transcription factor family that are induced by stress, such as DREB, ERF, WRKY, MYB, bZIP, helix-loop-helix, and NAC. In addition to being engaged in responses to drought specifically, ABA also interacts with responses to cold and salt stress [91].
-
THE INTERPLAY OF BIOTIC AND ABIOTIC STRESS SIGNALING:
-
To combat biotic and abiotic stressors, plants have evolved several strategies. The molecular pathways linked to stress tolerance have traditionally been investigated separately for each stress. As a result, our understanding of the signaling pathways involved in both biotic and abiotic stress responses is still limited. Recent research on chickpeas revealed that plant responses to the fungus Ascochyta blight were more comparable to high- salinity stress than to drought and cold conditions [92]. This is supported by the fact that the R2R3MYB transcription factor-encoding myb1 (SlAIM1) gene from tomato (Solanum lycopersicum) was activated by pathogens, plant hormones, salt, and oxidative stress [93]. Additionally, RNA interference-mediated SlAIM1 silencing enhanced sensitivity to salt and oxidative stress as well as susceptibility to the necrotrophic fungus Botrytis cinerea. SlAIM1 was also expressed ectopically, resulting in enhanced salinity and oxidative stress tolerance [94]. As a result, it was hypothesized that SlAIM1 controls a transmembrane ion flow, indicating an early response to abiotic stress and pathogen infection, maybe before hypersensitive cell death and necrosis.
Misregulation of ion fluxes can affect plant tolerance to necrotrophic infection or abiotic stress [95]. According to emerging data, hormone signaling pathways such as those mediated by jasmonic acid, abscisic acid, ethylene, and salicylic acid are critical to the interaction of abiotic and biotic stress responses [96]. Several transcription factors and kinases have been identified as key candidates for stress signaling pathway cross-talk in recent investigations. Mitogen-activated protein kinases (MAPKs) are involved in the signaling of developmental, hormonal, abiotic, and biotic stress [97]. The activation of MAPK cascade components by more than one form of stress shows that MAPK cascades operate as a junction for several abiotic and biotic stress signaling pathways. Furthermore, because the Arabidopsis genome is known to include roughly 20 MAPKs, 10 MAPKKs, and 60 MAPKKKs, signals detected by the 60 MAPKKKs must be conveyed via 10 MAPKKs to the 20 MAPKs, opening up the possibility of crosstalk between various stress signals.
Spatial and temporal expression patterns based on cell biology analysis together with biochemical identification of the signaling components are required to establish specificity or crosstalk of the signaling pathways [98]. Deeper knowledge of signaling pathways, specificity, and cross-talk should be focused on in the future years, with the further development and inclusion of "omics" technologies and computational methodologies. Currently, just a few routes and their components have been identified. Plants, on the other hand, confront and respond to a plethora of stimuli (both biotic and abiotic) in nature.
ROLE OF MicroRNA AND OTHER NOVEL GENES IN PLANT STRESS TOLERANCE:
The discovery and functional connection of microRNAs (miRNAs) has resulted in a significant new study topic in the previously unknown realm of non-coding RNAs [99]. MiRNAs are endogenous, short non-protein coding RNAs of 21-24 nucleotides that have lately emerged as significant regulators of gene expression [100]. These control target gene expression by catalyzing posttranslational gene silencing [101] or inhibition of translation [102]. MicroRNA targets include transcription factors and other regulatory proteins involved in plant growth or signal transduction. Micro-RNA (miRNA) study has recently shown a link between miRNAs and plant stress responses [103]. However, the connection between microRNAs and stress response is just now being investigated. Abiotic stressors either up or down-regulate many miRNAs, suggesting that they are involved in stress- responsive gene expression and stress adaption, influencing several cellular and physiological processes [104].
Sunkar and Zhu (2004) discovered new and abiotic stress-regulated miRNAs in Arabidopsis seedlings subjected to dehydration, salt, or cold stress and revealed differential expression of several of the discovered miRNAs. Zhao et al. (2007) investigated the transcript expression patterns of miRNAs in drought-stressed rice (Oryza sativa). Drought-induced expression of miR -169g and miR 393 was verified using microarray expression profiling, which revealed that miR -169g was more abundant in roots than in shoots. Sequence analysis indicated the presence of two proximal DREs (dehydration-responsive elements) upstream of MiR- 169g, indicating that CBF/DREBs regulate miR-169g transcript expression.
Sunkar et al. (2006) demonstrated the role of miRNA in oxidative stress responses by targeting cytosolic and chloroplastic superoxide dismutases, which detoxify superoxide radicals. In response to oxidative stress, miR 398 transcript expression was down-regulated, resulting in posttranscriptional accumulation of SOD mRNA and hence oxidative stress tolerance. Furthermore, transgenic Arabidopsis plants overexpressing a miR 398-resistant variant of SOD accumulated more mRNA than ordinary plants,
making them far more resistant to strong light, heavy metals, and other oxidative stressors. Arabidopsis has been found to accumulate miR159 in response to ABA, drought stress, and gibberellic acid (GA), and the miRNA has been predicted to target four MYB transcription factors [105]. Patade and Suprasanna (2010) have studied the transcript expression of mature miR159 in sugarcane in response to short- and long-term salt and PEG-induced osmotic stress. There was no change in mature transcript levels of miR159 in response to long-term (15 days) NaCl or iso-osmotic (-0.7 MPa) PEG stress. Short-term (up to 24 h) salt or PEG stressors, on the other hand, raised the mature miRNA transcript level compared to the control. The gene's early activation during the brief treatments suggests that it is involved in the regulation of genes involved in stress perception and/or signaling. Zhou et al. (2008) created a computational transcriptome-based method for annotating stress-inducible miRNAs in plants. The presence of numerous recognized stress-responsive cis-regulatory elements was discovered in the promoters of the miRNA genes, which was surprising. Continued efforts are required to determine the whole range of miRNAs and other small RNAs involved in stress regulatory pathways. The discovery and functional validation of stress-regulated short RNAs, including miRNAs, will aid in the development of new techniques for stress tolerance [106].
ABIOTIC STRESS TOLERANCE ENHANCEMENT STRATEGIES:
Screening of various genetic backgrounds has been used in many ways for enhancing abiotic stress tolerance in a specific genetic background. Resources, extensive crossing, and subsequent recurrent backcrossing; identification and selection of the primary conditioning genes using linkage mapping and quantitative trait loci (QTL) analysis; mutant population creation and screening, and transgenic insertion of new genes. Despite some success in transplanting tolerance features from wild relatives [107] into crop cultivars, In general, relatively little success has been observed in introducing high abiotic tolerance into top germplasm [108].
As previously stated, breeding for or inducing abiotic stress tolerance characteristics is virtually always constrained by the genetic complexity of the underlying processes as well as possible interaction among genetic determinants. Additionally, additional environmental conditions, plant development stage, inadequate or irreproducible selection procedures, and the logistical restrictions of physiological screening of large breeding populations on a field scale may all influence the differential selection of specific stress [109]. In this sense, identifying discrete chromosomal areas with a significant influence on a certain tolerance trait by quantitative trait loci (QTL) mapping and marker-assisted selection remains a helpful alternative for many breeding efforts [110]. This is especially true when the entire genome is unknown and no putative tolerance genes are identified.
Reliable and realistic screening approaches are necessary for the proper selection of the associated phenotype. However, the uniformity and reliability of field-based screening may be compromised due to variability in stress throughout the site (e.g., boron or salt level) as well as potential compounding environmental conditions (i.e. disease, rainfall, temperature). Furthermore, when the starting material is genetically diverse, variability among genetic backgrounds may impair the capacity to correctly pick the most superior or diverse tolerances. Alternatively, cellular-based mutant production and subsequent selection under-regulated in vitro conditions provide a mechanism for rapidly screening large populations with homogenous backgrounds for unique serendipitous tolerance alterations. Subsequent field screening verifies that the tolerance trait performs well in the presence of the previously indicated external possibly mitigating circumstances. Unsurprisingly, this strategy has sparked considerable interest in choosing abiotic stress tolerances in a variety of crop species [111].
TRANSGENIC METHODS FOR ENGINEERING TOLERANCE:
Many genes are connected to many pathways and processes, including stress sensing and signaling, and contribute to molecular, biochemical, cellular, physiological, and morphological changes in response to plant stress [112]. Stress-sensitive sensitive genes are those that reduce the impact of stress and lead to changes in the cellular environment and plant tolerance. The gene products are divided into three categories: those that directly protect plant cells from stress, those that participate in signaling cascades and transcriptional regulation, and those that participate in water and ion absorption and transport.
Engineering metabolic and stress-signaling pathways to develop stress-tolerant crops is one of the agricultural research's main objectives [113]. Stress-inducible genes have been used in the genetic transformation to better understand their functional involvement in the tolerance response and, eventually, to increase the tolerance trait in the target genotype [114]. By far the majority of this research has been single-gene in nature. Transactions within well-known multigenic pathways, most of which are engaged in signaling and regulatory pathways, or effector genes that code for enzymes that catalyze the production of structural and functional components. When determining the effectiveness of a transformation experiment, one of the most important factors to examine is whether the transgenic plants express a greater amount of the transgene (i.e. an osmoprotectant or a protein) exclusively under stress circumstances [115]. Tolerance/stress-induced mechanisms may be energy and nucleic acid-demanding, diverting important resources away from normal development processes, hence particular inducible promoters are employed rather than constitutive promoters [116].
Transgenic rice plants containing choline oxidase (codA), d-pyrroline-5-carboxylate synthase (P5CS), LEA protein group 3 (HVA1), alcohol dehydrogenase (ADH), and pyruvate decarboxylase (PDC) genes, for example, shown drought tolerance [117]. Potato and rice [118] modified with trehalose production genes (Yeo et al. 2000 and Garg et al. 2002, respectively) demonstrated drought tolerance (in the case of potato) as well as salt, drought, and low-temperature stress tolerance (in the case of rice). Tobacco plants that were transformed with ectoine production genes from the halophilic bacteria Halomonas elongate demonstrated improved salt tolerance. Furthermore, transformation with sorbitol (Sheveleva et al. 1997) or mannitol (Shen et al. 1997) genes led to higher osmolyte accumulation and tolerance to high salinity. Overexpression of genes encoding the enzymes pyrroline-5-
carboxylate (P5C) synthetase (P5CS) and P5CR led to proline overproduction and improved abiotic stress tolerance [119]. P5CS overexpression in transgenic tobacco increased seedling germination and growth under salt stress. Transgenic petunia plants transformed with the Arabidopsis P5CS gene were more drought resistant than control plants [120].
Another technique for improving drought, salt, and freezing tolerance are to increase glycine betaine (GB) production in transgenic plants by employing genes that encode for enzymes (choline monooxygenase, betaine aldehyde dehydrogenase, and choline oxidase) in GB biosynthesis [121]. Transgenic rice plants expressing the codA (choline oxidase) gene developed normally after recovering from an early growth inhibition caused by salt and low-temperature stress [122]. Other plants that have been genetically modified for salt, drought, cold, and heat tolerance via GBS accumulation include: A. Brassica napus, Brassica juncea, Gossypium hirsutum, Lycopersicon esculentum, Nicotiana tabacum, Solanum tuberosum, and Zea mays are all members of the Brassica genus [123].
Trehalose is a non-reducing disaccharide that acts as an osmoprotectant [124]. Transgenic plants overexpressing trehalose biosynthesis genes showed enhanced tolerance to a variety of abiotic stressors [125]. To provide tolerance to various abiotic conditions, a stress-inducible promoter was used to overexpress Escherichia coli trehalose biosynthetic genes (otsA and otsB) as a fusion gene (TPSP) in rice [126]. The TPSP fusion gene is useful in that both genes may be introduced into the rice genome at the same time, resulting in enhanced catalytic efficiency for trehalose production [127].
Other osmolytes, including mannitol, fructans, ononitol, proline, glycine betaine, and ectoine, have shown promise in genetic engineering attempts for producing resistant genotypes [128]. Overproduction of suitable solutes should be done under stress- inducible and/or tissue-specific control to prevent burdening the plant's metabolic machinery and perhaps reducing pleiotropic benefits. Furthermore, the production of osmolytes should be directed toward the chloroplast by inserting a signal sequence in front of the engineered enzymes [129].
Abiotic stress, as previously stated, causes a rise in reactive oxygen species, which may be harmful to normal cellular activities. As a result, multiple oxidative-stress-related genes have been used to create transgenic plants that are resistant to a variety of pressures [130]. Transgenic tobacco plants overexpressing chloroplastic Cu/Zn-SOD, for example, demonstrated improved tolerance to oxidative stress produced by salt exposure [131]. Transgenic alfalfa (Medicago sativa) plants expressing Mn-SOD displayed less water-deficit stress damage, as measured by chlorophyll fluorescence, electrolyte leakage, and regrowth [132]. Simultaneous expression of three antioxidant enzyme genes: The presence of copper-zinc superoxide dismutase, ascorbate peroxidase, and dehydroascorbate reductase in tobacco chloroplasts imparted increased tolerance to oxidative stressors produced by paraquat and salt [133]. Similarly, AtNDPK2 overexpression effectively regulated oxidative stress. Sweet potato stress produced by diverse environmental pressures is reduced by increased antioxidant enzyme activities such as peroxidase, ascorbate peroxidase, and catalase [134]. Thus, targeting detoxification pathways as a strategy for getting plants with diverse stress-tolerance features appears promising.
Transgenic modification of detoxification pathways by overexpression of oxidative protection genes like glutathione peroxidase, superoxide dismutase, ascorbate peroxidases, and glutathione reductases is a current topic of research. Nicotiana PK1 gene (regulatory protein NPK1) constitutive expression improved freeze, heat, and salinity tolerance in transgenic maize plants [135]. Shou et al. (2004a) found that constitutively expressing a tobacco MAPKKK (NPK1) in maize improved drought tolerance. The transgenic maize plants retained considerably greater rates of photosynthesis, suggesting that NPK1 generated a mechanism that protected photosynthesis machinery from dehydration damage.
To sustain normal metabolic activity under salt stress, tolerant plant cells must maintain high K+ (100-200 mM) and low Na+ (less than 1 mM) levels. Helping plants re-establish homeostasis in harsh settings, restoring both ionic and osmotic equilibrium, is essential for increasing resistance to salt stress. This concept remains a key effort to improve salt tolerance in plants by genetic engineering, to achieve Na+ excretion or vacuolar storage. A variety of abiotic stress-tolerant transgenic plants have been created by raising the cellular levels of proteins that affect transport processes (such as vacuolar antiporter proteins). For example, Arabidopsis AtSOS has been revealed to encode a plasma membrane Na+ /H+antiporter (NHX) with strong sequence similarities to bacteria and fungal antiporters [136]. In Arabidopsis, constitutive activation of the vacuolar Na+/H+ antiporter (NHX1) or AVP1 (A. thaliana vacuolar H+ translocating pyrophosphatase) gene accelerated the pumping of Na+ into the vacuole and enhanced both accumulation and Na+ tolerance [137]. By lowering cytosolic Na+ concentrations, more effective sequestration of these ions to the vacuole might increase tissue tolerance to salt. The significance of Na+ sequestration in salt tolerance has been proven further in transgenic tomato plants overexpressing the AtNHX1 gene [138]. In addition, a vacuolar chloride channel gene, AtCLCd, implicated in cation detoxification, has been cloned and demonstrated to confer salt tolerance in Arabidopsis. The Salt Overly Sensitive 1 (SOS1) gene was upregulated in Arabidopsis, resulting in a higher proton motive force required for enhanced Na+ /H+ antiporter activity [139].
In addition to the single gene strategy, multiple stress tolerance can be accomplished by targeting a stress-inducible signal transduction molecule and/or transcription factor [140]. Transcription factors are vital in the development of stress tolerance, which eventually benefits agricultural and environmental operations [141]. The plant's reaction to abiotic stress is mediated by a wide number of transcription factors [142]. The majority of them are members of big transcription factor families such as AP2/ERF, bZIP, NAC, MYB, MYC, Cys2His2 zinc-finger, and WRKY. As a consequence, overexpression of the functionally conserved At-DBF2 gene resulted in Arabidopsis exhibiting a broad and high level of multiple stress tolerance [143]. Overexpression of the calcineurin gene, a Ca 2+/calmodulin-dependent protein phosphatase gene formally recognized as being involved in salt-stress signal transduction in yeast, resulted in salt-stress-tolerant tobacco plants [144].
Some stress-responsive genes may share transcription factors, as seen by the considerable overlap in gene expression patterns caused by drought and cold stress [145]. Transgenic plants have been able to activate stress-induced genes by overexpressing one or more transcription factors that identify regulatory regions of these genes. The transcription factor DREB1A uniquely interacts with the DRE in Arabidopsis and stimulates the expression of stress tolerance genes [146]. Through robust constitutive expression of the stress-inducible genes, CaMV 35S promoter-driven overexpression of DREB1A cDNA in transgenic Arabidopsis plants afforded resistance to salt, cold, and drought stress [147].
The transcription factors involved in the ABA-dependent (such as NAC, AREB / ABF, and MYB) and ABA-independent (AP2 / ERF gene) stress response pathways control a cascade of downstream genes and events that improve drought tolerance. Transforming crops with such transcription factor genes should have a greater impact on drought tolerance development [148]. Overexpression of Arabidopsis CBF1 (CRT / DRE) cDNA in tomatoes increased tolerance to salt, cold, and drought stress; nevertheless, the plants had a dwarf phenotype and had a lower fruit set and seed quantity [149]. Alfin1 (transcriptional regulator) overexpression in alfalfa plants resulted in salt tolerance via controlled endogenous MsPRP2 (NaCl-inducible gene) mRNA levels [150].
TRANSGENIC APPROACHES IN THE FUTURE:
Changing the expression levels of native genes or integrating foreign genes for osmolytes, ion transporters, transcription factors, and other signaling molecules is the current plant genetic engineering technique for producing salt-stress-tolerant transgenic plants. The development of global transcription profiling has revealed that many more genes are concurrently up and down- regulated in response to salt stress. This second group of genes produces proteins involved in the control of transcriptional and translational machinery, each of which has a unique function in modulating the salt stress response. The coordinated induction and activation of many RNA binding proteins, ribosomal genes, helicases, cyclophilins, F-box proteins, dynamin-like proteins, translation initiation, and elongation factors appear to be involved in salt stress tolerance. The cellular functioning of these genes should also be explored to determine their suitability for targeted transgenic techniques [151].
The assessment of genetically altered salt tolerant transgenic lines necessitates critical, rigorous, and exhaustive testing [152]. Under regulated saline and non-saline treatment circumstances, fourth or fifth-generation genotypes should be examined alongside parental (wild-type) lines. Validation should not end in the laboratory or greenhouse, because quantitative growth measurements are required throughout the plant's life cycle in the field.
CONCLUSIONS AND FUTURE PROSPECTS:
Significant progress has been made in our knowledge of the complicated processes driving abiotic stress tolerance in agricultural plants during the last decade. However, we are still a long way from pinpointing the exact set of genes responsible for tolerance to a certain abiotic stress scenario. This scenario is compounded by the fact that plants must deal with several biotic and abiotic stimuli at the same time. Our quest to comprehend these complicated pathways is continuing, and the recent advent of new technologies for high-throughput genotyping and phenotyping provides us with new hope. In the near future, a thorough understanding of physiological and molecular mechanisms, particularly signaling cascades in response to abiotic stressors on plants, will aid in manipulating vulnerable crop plants and increasing agricultural productivity.
REFERENCES:
[1] "Abiotic Stress". Biology Online. Archived from the original on 13 June 2008. Retrieved 2008-05-04. [2] Vinebrooke, Rolf D.; et al. (2004). "Impacts of multiple stressors on biodiversity and ecosystem functioning: the role of species co- tolerance". OIKOS. 104 (3):451457 [3] Vorasoot N, Songsri P, Akkasaeng C, Jogloy S, Patanothai A (2003) Effect of water stress on yield and agronomic characters of peanut. Songklanakarin J Sci Technol 25(3):283288 [4] Jaleel CA, Manivannan P, Wahid A, Farooq M, Somasundaram R, Panneerselvam R (2009) Drought stress in plants: a review on morphological characteristics and pigments composition. Int J Agric Biol 11:100105 [5] Collins NC, Tardieu F, Tuberosa R (2008) Quantitative trait loci and crop performance under abiotic stress: where do we stand? Plant Physiol 147:469 486 [6] Flowers TJ, Yeo AR (1995) Breeding for salinity tolerance in crop plants: where next? Aust J Plant Physiol 22:875884 [7] Heidarvand L, Amiri RM (2010) What happens in plant molecular responses to cold stress? Acta Physiol Plant 32:419431 [8] Wang, W., Vinocur, B. and Altman, A., 2007. Plant responses to drought, salinity, and extreme temperatures towards genetic engineering for stress tolerance. Planta. 218:1-14. [9] Ashraf, M., Athar, H.R., Harris, P.J.C. and Kwon, T.R., 2008. Some prospective strategies for improving crop salt tolerance. Adv. Agron., 97: 45110. [10] Buchanan, B.B., Gruissem, W. and Jones, R.L., 2000. Biochemistry and molecular biology of plants. American Soc. Plant Physiologists. Rockville.Maryland. USA FAO. (1985). Production year book, 39. Roam: FAO
[11] Levitt, J. (1980) Responses of plants to environmental stresses. II. Water, radiation, salt, and other stresses. New York: Academic Press. [12] Quinn, P.J. (1988) Effects of temperature on cell membranes. Symposia of Society for Experimental Biology 42: 237258. [13] Levitt, J. (1980) Responses of plants to environmental stresses. II. Water, radiation, salt, and other stresses. New York: Academic Press. [14] Fowler, D.B., Dvorak, J., Gusta, L.V. (1977) Comparative Cold Hardiness of Several Triticum species and Secale cereale L. Crop Science 17: 941943. [15] Hall, A.E. (2001) Crop Responses to the Environment. Boca Raton, Florida: CRC Press. [16] Munns, R. (2002) Comparative physiology of salt and water stress. Plant, Cell and Environment 25(2): 239250. [17] Khajeh-Hosseini, M., Powell, A.A., Bingham, I.J. (2003) The interaction between salinity stress and seed vigour during germination of soybean seeds.Seed Science and Technology 31(3): 715725.
[18] Kozlowski, T.T., Pallardy, S.G. (1997) Growth control in woody plants. San Diego: Academic Press. [19] Herrera-Estrella, L., Guevara-Garc´a, A., Lo´pez-Bucio, J. (1999) Heavy metal adaptation. In Encyclopedia of Life Sciences, 15. London: Macmillan Publishers. [20] Munns R, Tester M (2008) Mechanisms of salinity tolerance. Annu Rev Plant Biol 59 [21] Flowers TJ, Dalmond D (1992) Protein synthesis in halophytes: the influence of potassium, sodium, and magnesium in vitro. Plant Soil 146:153161 [22] Munns R, Tester M (2008) Mechanisms of salinity tolerance. Annu Rev Plant Biol 59:6516 [23] Silva P, Facanha AR, Tavares RM, Geros H (2010) Role of tonoplast proton pumps and Na + /H + antiport system in salt tolerance of Populus euphratica oliv. J Plant Growth Regul 29:2334 [24] Munns R, Tester M (2008) Mechanisms of salinity tolerance. Annu Rev Plant Biol 59:65 [25] RodrÃguez-Rosales MP, Gálvez FJ, Huertas R, Aranda MN, Baghour M, Cagnac O, Venema K (2009) Plant NHX cation/proton antiporters. Plant Signal Behav 4(4):265276 [26] Shi H, Ishitani M, Kim C, Zhu JK (2000) The Arabidopsis thaliana salt tolerance gene SOS I encodes a putative Na /H + antiporter. Proc Natl Acad Sci USA 97:68966901 [27] Gaxiola RA, Li J, Unurraga S, Dang LM, Allen GJ, Alper SL, Fink GR (2001) Drought- and salt-tolerant plants result from overexpression of the AVP1 H + -pump. Proc Natl Acad Sci USA 98:1144411449 [28] Leidi EO, Barragán V, Rubio L, El-Hamdaoui A, Ruiz MT, Cubero B, Fernández JA, Bressan RA, Hasegawa PM, Quintero FJ, Pardo JM (2010) The AtNHX1 exchanger mediates potassium compartmentation in vacuoles of transgenic tomato. Plant J 61(3):495506 [29] Valliyodan B, Nguyen HT (2006) Understanding regulatory networks and engineering for enhanced drought tolerance in plants. Curr Opin Plant Biol 9:17 [30] Ford CW (1984) AccuThe emulation of low molecular weight solutes in water stress tropical legumes. Phytochemistry 22:875884 [31] Delauney AJ, Verma DP (1993) Proline biosynthesis and osmoregulation in plants. Plant J 4:215223 [32] Chen THH, Murata N (2008) Glycinebetaine: an effective protectant against abiotic stress in plants. Trends Plant Sci 13(9):499505 [33] Krishnan N, Dickman MB, Becker DF (2008) Proline modulates the intracellular redox environment and protects mammalian cells against oxidative stress. Free Radic Biol Med 44(4):671681 [34] Bohnert HJ, Shen B (1999) Transformation and compatible solutes. Sci Hortic 78: [35] Bensen R, Castiglioni P, Korte J, Bell E, Hinchey B, Loida P, Ahrens J (2008) Transgenic plants with increased glycine-betaine. United States Patent 7410800. Application Number:10/839092 [36] Delauney AJ, Verma DP (1993) Proline biosynthesis and osmoregulation in plants. Plant J 4:215223 [37] Suprasanna P, Teixeira da Silva JA, Bapat VA (2005) Plant abiotic stress, sugars, and transgenics: a perspective. In: Teixeira da Silva JA (ed) Floriculture, ornamental and plant biotechnology: advances and topical issues, 1st edn. Global Science Publishers, London, UK, pp 8693 [38] Suprasanna P (2003) Building stress tolerance through over-producing trehalose in transgenic plants. Trends Plant Sci 8(8):355357 [39] Cortina C, Culianez-Macia FA (2005) Tomato abiotic stress enhanced tolerance by trehalose biosynthesis. Plant Sci 169:7582 [40] Prabhavathi VR, Rajam MV (2007) Polyamine accumulation in transgenic eggplant enhances tolerance to multiple abiotic stresses and fungal resistance.Plant Biotechnol 24:273282
[41] Verdoy D, Coba de la peña T, Redondo FJ, Lucas MM, Pueyo JJ (2006) Transgenic Medicago truncatula plants that accumulate proline display nitrogen- fi xing activity with enhanced tolerance to osmotic stress. Plant Cell Environ 29:19131923 [42] Su J, Wu R (2004) Stress-inducible synthesis of proline in transgenic rice confers faster growth under stress conditions than that with constitutive synthesis. Plant Sci 166(4):941948 [43] Thomashow MF (2010) Molecular basis of plant cold acclimation: insights gained from studying the CBF cold response pathway. Plant Physiol 154:571 577 [44] Li C, Puhakainen T, Welling A, Viherä-Aarnio A, Ernstsen A, Junttila O, Heino P, Palva ET (2002) Cold acclimation in silver birch (Betula pendula ).Development of freezing tolerance in different tissues and climatic ecotypes. Physiol Plant 116:478488
[45] Li C, Junttila O, Heino P, Palva ET (2003) Different responses of northern and southern ecotyps of Betula pendula to exogenous ABA application. Tree Physiol 23:481487 [46] Welling A, Rinne P, Vihera-Aarnio A, Kontunen-Soppela S, Heino P, Palva ET (2004) Photoperiod and temperature differentially regulate the expression of two dehydrin genes during overwintering of birch (Betula pubescens Ehrh.). J Exp Bot 55:507516 [47] Zhu B, Coleman GD (2001) Phytochrome-mediated photoperiod perception, shoot growth, glutamine, calcium, and protein phosphorylation influence the activity of the poplar bark storage protein gene promoter (bspA). Plant Physiol 126:34235 [48] Uemura M, Warren G, Steponkus PL (2003) Freezing sensitivity in the sfr4 mutant of Arabidopsis is due to low sugar content and is manifested by loss of osmotic responsiveness. Plant Physiol 131:18001807 [49] Stitt M, Hurry V (2002) A plant for all seasons: alterations in photosynthetic carbon metabolism during cold a Low-temperature arabidopsis. Curr Opin Plant Biol 5:199206 [50] Krebs JA, Wu Y, Chang H-S, Zhu T, Wang X, Harpecold-induced transcriptome changes for Arabidopsis in response to salt, osmotic, and cold stress.Plant Physiol 130:21292141
[51] Thomashow MF (2010) Molecular basis of plant cold acclimation: insights gained from studying the CBF cold response pathway. Plant Physiol 154:571 577 [52] Chinnusamy V, Zhu J, Zhu JK (2006) Gene regulation during cold acclimation in plants. Physiol Plant 126:5261 [53] Thomashow MF (2010) Molecular basis of plant cold acclimation: insights gained from studying the CBF cold response pathway. Plant Physiol 154:571 577 [54] Gilmour SJ, Zarka DG, Stockinger EJ, Salazar MP, Houghton JM, Thomashow MF (1998) Low temperature regulation of the Arabidopsis CBF family of AP2 transcriptional activators as an early step in cold induced COR gene expression. Plant J 16:43 [55] Riechmann JL, Heard J, Martin G, Reuber L, Jiang CZ, Keddie J, Adam L, Pineda O, Ratcliffe OJ, Samaha RR, Creelman R, Pilgrim M, Broun P, Zhang JZ, Ghandehari D, Sherman BK, Yu GL (2000) Arabidopsis transcription factors: genome-wide comparative analysis among eukaryotes. Science 290:210 [56] Stockinger EJ, Gilmour SJ, Thomashow MF (1997) Arabidopsis thaliana CBF1 encodes an AP2 domain-containing a transcriptional activator that binds to the C-repeat/DRE, a cis-acting DNA regulatory element that stimulates transcription in response to low temperature and water deficit. Proc Natl Acad Sci USA 94:10351040 [57] Maruyama K, Sakuma Y, Kasuga M, Ito Y, Seki M, Goda H, Shimada Y, Yoshida S, Shinozaki K, Yamaguchi Shinozaki K (2004) Identification of cold- inducible downstream genes of the Arabidopsis DREB1A/CBF3 transcriptional factor using two microarray systems. Plant J 38:982993 [58] Jaglo-Ottosen KR, Gilmour SJ, Zarka DG, Schabenberger O, Thomashow MF (1998) Arabidopsis CBF1 overexpression induces COR genes and enhances freezing tolerance. Science 280:104106 [59] Kaplan F, Kopka J, Haskell DW, Zhao W, Schiller KC, Gatzke N, Sung DY, Guy CL (2004) Exploring the temperature stress metabolome of Arabidopsis.Plant Physiol 136:41594168
[60] Steponkus PL, Uemura M, Joseph RA, Gilmour SJ, Thomashow MF (1998) Mode of action of the COR15a gene on the freezing tolerance of Arabidopsis thaliana. Proc Natl Acad Sci USA 95:1457014575 [61] Sangwan V, Orvar BL, Beyerly J, Hirt H, Dhindsa RS (2002) Opposite changes in membrane fluidity mimic cold and heat stress activation of distinct plant MAP kinase pathways. Plant J 31:629638 [62] Knight H, Trewavas AJ, Knight MR (1996) Cold calcium signaling in Arabidopsis involves two cellular pools and a change in calcium signature after acclimation. Plant Cell 8:489503 [63] Shinozaki K, Yamaguchi-Shinozaki K (1996) Molecular responses to drought and cold stress. Curr Opin Biotechnol 7:161167 [64] Jaglo-Ottosen KR, Gilmour SJ, Zarka DG, Schabenberger O, Thomashow MF (1998) Arabidopsis CBF1 overexpression induces COR genes and enhances freezing tolerance. Science 280:104106 [65] Kasuga M, Liu Q, Miura S, Yamaguchi-Shinozaki K, Shinozaki K (1999) Improving plant drought, salt, and freezing tolerance by gene transfer of a single stress-inducible transcription factor. Nat Biotechnol 17: 287291 [66] Tahtiharju S, Sangwan V, Monroy AF, Dhindsa RS, Borg M (1997) The induction of kin genes in cold-acclimating Arabidopsis thaliana: evidence of a role for calcium. Planta 203:4424 [67] Xu J, Zhang Y, Guan Z, Wei W, Han L, Chai T (2008) Expression and function of two dehydrins under environmental stresses in Brassica juncea L. Mol Breed 21(4):431438 [68] Sarhadi E, Mahfoozi S, Hosseini SA, Salekdeh GH (2010) Cold acclimation proteome analysis reveals a close link between the up-regulation of low- temperature associated proteins and vernalization fulfillment. J Proteome Res 9(11):56585667 [69] Apel K, Hirt H (2004) Reactive oxygen species: metabolism, oxidative stress, and signal transduction. Annu Rev Plant Biol 55:373399 [70] Einset J, Winge P, Bones A (2007) ROS signaling pathways in chilling stress. Plant Signal Behav 2(5):365367 [71] Jones A (2000) Does the plant mitochondrion integrate cellular stress and regulate programmed cell death? Trends Plant Sci 5:273278 [72] Imlay JA (2003) Pathways of oxidative damage. Annu Rev Microbiol 57:395418 [73] Vranova E, Inze D, Breusegem F (2002) Signal transduction during oxidative stress. J Exp Bot 53: 12271236 [74] Desikan R, Cheung MK, Bright J, Henson D, Hancock JT, Neill SJ (2004) ABA, hydrogen peroxide and nitric oxide signaling in stomatal guard cells. J Exp Bot 55:205212 [75] Krishnan N, Dickman MB, Becker DF (2008) Proline modulates the intracellular redox environment and protects mammalian cells against oxidative stress. Free Radic Biol Med 44(4):671681 [76] Roxas VP, Lodhi SA, Garrett DK, Mahan JR, Allen RD (2000) Stress tolerance in transgenic tobacco seedlings that overexpress Glutathione S-Transferase/ Glutathione Peroxidase. Plant Cell Physiol 41(11): 12291234
[77] Lu YY, Deng XP, Kwak SS (2010) Overexpression of CuZn superoxide dismutase (CuZn SOD) and ascorbate peroxidase (APX) in transgenic sweet potato enhances tolerance and recovery from drought stress. Afr J Biotechnol 9(49):83788391 [78] Baier M, Noctor G, Foyer CH, Dietz KJ (2000) Antisense suppression of 2-Cysteine peroxiredoxin in Arabidopsis specifically enhances the activities and expression of enzymes associated with ascorbate metabolism but not glutathione metabolism. Plant Physiol 124(2): 823832 [79] Sugie A, Naydenov N, Mizuno N, Nakamura C, Takumi S (2006) Overexpression of wheat alternative oxidase gene Waox1a alters respiration capacity and response to reactive oxygen species under low temperature in transgenic Arabidopsis. Genes Genet Syst 81: 3493 [80] Zhu J, Dong CH, Zhu JK (2007) Interplay between cold-responsive gene regulation, metabolism, and RNA processing during plant cold acclimation.Curr Opin Plant Biol 10:290295
[81] Snedden WA, Fromm H (2001) Calmodulin as a versatile calcium signal transducer in plants. New Phytol 151:3566 [82] Zhang T, Wang Q, Chen X, Tian C, Wang X, Xing T, Li Y, Wang Y (2005) Cloning and biochemical properties of CDPK gene OsCDPK14 from rice. J Plant Physiol 162(10):11491159 [83] Saijo Y, Hata S, Kyozuka J, Shimamoto K, Izui K (2000) Over-expression of a single Ca 2+ -dependent protein kinase confers both cold and salt/drought tolerance on rice plants. Plant J 23:319327 [84] Mantri NL, Pang ECK, Ford R (2010b) Molecular biology for stress management. In: Yadav SS, McNeil DN, Weeden N, Patil SS (eds) Climate change and management of cool season grain legume crops. Springer, Heidelberg, pp 377408. ISBN 978-90-481-3708-4 [85] Shinozaki K, Yamaguchi-Shinozaki K (2000) Molecular response to dehydration and low temperature: differences and cross-talk between two stress signaling pathways. Curr Opin Plant Biol 3:217223 [86] Seki M, Okamoto M, Matsui A, Kim JM, Kurihara Y, Ishida J, Moosawa T, Kawashima M, Kim T, Shinozaki K (2009) Microarray analysis for studying the abiotic stress responses in plants. Mol Tech Crop Improve 3:333355 [87] Munns R, Tester M (2008) Mechanisms of salinity tolerance. Annu Rev Plant Biol 59:651 [88] Pearce R (2001) Plant freezing and damage. Ann Bot 87:417424 [89] Baima S, Possenti M, Matteucci A, Wisman E, Altamura M, Ruberti I, Morelli G (2001) The Arabidopsis ATHB-8 HD-Zip protein acts as a differentiation-promoting transcription factor of the vascular meristems. Plant Physiol 126(2):643655 [90] Kizis D, Lumbreras V, Pagès M (2001) Role of AP2/ EREBP transcription factors in gene regulation during abiotic stress. FEBS Lett 498(23):187189 [91] Seki M, Narusaka M, Ishida J, Nanjo T, Fujita M, Oono Y, Kamiya A, Nakajima M, Enju A, Sakurai T, Satou M, Akiyama K, Taji T, Yamaguchi-Shinozaki K, Carninci P, Kawai J, Hayashizaki Y, Shinozaki K (2002) Monitoring expression profile of 7000 Arabidopsis genes under drought, cold and high-salinity stresses using a full-length cDNA microarray. Plant J 31:279292
[92] Mantri NL, Ford R, Coram TE, Pang ECK (2010a) Evidence of unique and shared responses to major biotic and abiotic stresses in chickpea. Environ Exp Bot 69(3):286292 [93] Abuqamar S, Luo H, Laluk K, Mickelbart MV, Mengiste T (2009) Crosstalk between biotic and abiotic stress responses in tomato is mediated by the AIM1 transcription factor. Plant J 58(2):347360 [94] Abuqamar S, Luo H, Laluk K, Mickelbart MV, Mengiste T (2009) Crosstalk between biotic and abiotic stress responses in tomato is mediated by the AIM1 transcription factor. Plant J 58(2):347360 [95] Abuqamar S, Luo H, Laluk K, Mickelbart MV, Mengiste T (2009) Crosstalk between biotic and abiotic stress responses in tomato is mediated by the AIM1 transcription factor. Plant J 58(2):347360 [96] Fujita M, Fujita Y, Noutoshi Y, Takahashi F, Narusaka Y, Yamaguchi-Shinozaki K, Shinozaki K (2006) Crosstalk between abiotic and biotic stress responses: a current view from the points of convergence in the stress signaling networks. Curr Opin Plant Biol 9:436442 [97] Rodriguez MC, Petersen M, Mundy J (2010) Mitogen-activated protein kinase signaling in plants. Annu Rev Plant Biol 61:621649 [98] Chinnusamy V, Schumaker K, Zhu JK (2004) Molecular genetic perspectives on cross-talk and specificity in abiotic stress signaling in plants. J Exp Bot 55(395): 225236 [99] Reinhart BJ, Weinstein EG, Rhoades MW, Bartel B, Bartel DP (2002) microRNAs in plants. Genes Dev 16: 16161626 [100]Bartel D (2004) MicroRNAs: genomics, biogenesis, mechanism, and function. Cell 116(2):281297 [101]Palatnik JF, Allen E, Wu X, Schommer C, Schwab R, Carrington JC, Weigel D (2003) Control of leaf morphogenesis by microRNAs. Nature [102]Chen X (2004) A microRNA as a translational repressor of APETALA2 in Arabidopsis flower development. Science 303:20222025 [103]Patade VY, Suprasanna P (2010) Short-term salt, and PEG stresses regulate expression of MicroRNA, miR159 in sugarcane leaves. J Crop Sci Biotechnol 13(3): 177182 [104]Shukla LI, Chinnusamy V, Sunkar R (2008) The role of microRNAs and other endogenous small RNAs in plant stress responses. Biochim Biophys Acta 1779:743748 [105]Reyes JL, Chua NH (2007) ABA induction of miR159 controls transcript levels of two MYB factors during Arabidopsis seed germination. Plant J 49:592 606 [106]Katiyar-Agarwal S, Gao A, Vivian-Smith J (2007) A novel class of bacteria-induced small RNAs in Arabidopsis. Genes Dev 21:31233134 [107]Foolad MR, Zhang LP, Lin GY (2001) Identification and validation of QTLs for salt tolerance during vegetative growth in tomato by selective genotyping.Genome 44:444454
[108]Flowers TJ (2004) Improving crop salt tolerance. J Exp Bot 55:307319 [109]Flowers TJ (2004) Improving crop salt tolerance. J Exp Bot 55:307319 [110]Cuartero J, Bolarin MC, Moreno V, Pineda B (2010) Molecular tools for enhancing salinity tolerance in plants. In: Jain SM, Brar DS (eds) Molecular techniques in crop improvement. Springer, New York, pp 373405 [111]Suprasanna P, Sidha M, Bapat VA (2008) Integrated approaches of mutagenesis and in vitro selection for crop improvement. In: Kumar A, Shekhawat NS (eds) Plant tissue culture, molecular markers, and their role [112]Munns R, Tester M (2008) Mechanisms of salinity tolerance. Annu Rev Plant Biol [113]Cuartero J, Bolarin MC, Moreno V, Pineda B (2010) Molecular tools for enhancing salinity tolerance in plants. In: Jain SM, Brar DS (eds) Molecular techniques in crop improvement. Springer, New York, pp 373405 [114]Jewell MC, Campbell BC, Ian D (2010) Transgenic plants for abiotic stress resistance. In: Kole C et al (eds) Transgenic crop plants. Springer, Berlin, pp 67132 [115]Zhu JK (2001) Plant salt tolerance. Trends Plant Sci 6:6671 [116]Su J, Shen Q, Ho T-H, Wu R (1998) Dehydration-stress-regulated transgene expression in stably transformed rice plants. Plant Physiol 117:913922 [117]Soren KR, Ali K, Tyagi A, Tyagi V (2010) Recent developments in transgenics for abiotic stress tolerance in rice. Indian J Biotechnol 9:233251 [118]Yeo ET, Kwon HB, Han SE, Lee JT, Ryu JC, Byu MO (2000) Genetic engineering of drought resistant potato plants by introduction of the trehalose-6- phosphate synthase (TPS1) gene from Saccharomyces cerevisiae. Mol Cells 10:263268 [119]Szabados L, Savoure A (2010) Proline: a multifunctional amino acid. Trends Plant Sci 15(2):8997 [120]Yamada M, Morishita H, Urano K, Shiozaki N, Yamaguchi-Shinozaki K, Shinozaki K, Yoshiba Y (2005) Effects of free proline accumulation in petunias under drought stress. J Exp Bot 56:19751981 [121]Chen THH, Murata N (2008) Glycinebetaine: an effective protectant against abiotic stress in plants. Trends Plant Sci 13(9):499505 [122]Sakamoto A, Murata A, Murata N (1998) Metabolic engineering of rice leading to biosynthesis of glycine betaine and tolerance to salt and cold. Plant Mol Biol 38:10111019 [123]Chen THH, Murata N (2008) Glycinebetaine: an effective protectant against abiotic stress in plants. Trends Plant Sci 13(9):499505 [124]Goddijn OJM, van Dunn K (1999) Trehalose metabolism in plants. Trends Plant Sci 4:315319 [125]Almeida A, Cardoso L, Santos D, Torné J, Fevereiro P (2007) Trehalose and its applications in plant biotechnology. In Vitro Cell Dev Biol Plant 43(3):167177 [126]Garg AK, Kim JK, Owens TG, Ranwala AP, Choi YC, Kochian LV, Wu RJ (2002) Trehalose accumulation in rice plants confers high tolerance levels to different abiotic stresses. Proc Natl Acad Sci USA 99: 1589815903 [127]Almeida A, Cardoso L, Santos D, Torné J, Fevereiro P (2007) Trehalose and its applications in plant biotechnology. In Vitro Cell Dev Biol Plant 43(3):16717 [128]Suprasanna P, Teixeira da Silva JA, Bapat VA (2005) Plant abiotic stress, sugars, and transgenics: a perspective. In: Teixeira da Silva JA (ed) Floriculture, ornamental and plant biotechnology: advances and topical issues, 1st edn. Global Science Publishers, London, UK, pp 8693 [129]Shen B, Jensen RG, Bohnert HJ (1997) Increased resistance to oxidative stress in transgenic plants by targeting mannitol biosynthesis to chloroplasts.Plant Physiol 113:11771183
[130]Hussain TM, Thummala C, Mahamed H, Zafar S, Saleh BK, Rama GG (2008) Recent advances in salt stress biology a review. Biotechnol Mol Biol Rev 3(1):008013 [131]Bartel D (2001) Targeting detoxification pathways: an efficient approach to obtaining plants with multiple stress tolerance. Trends Plant Sci 6:284286 [132]McKersie BD, Bowley SR, Harjanto E, Leprince O (1996) Water-deficit tolerance and field performance of transgenic alfalfa overexpressing superoxidedismutase. Plant Physiol 111(4):11771181
[133]Lee YP, Kim SH, Bang JW (2007) Enhanced tolerance to oxidative stress in transgenic tobacco plants expressing three antioxidant enzymes in chloroplasts. Plant Cell Rep 26:591598 [134]Kim -H, Kim MD, Choi YI, Park S-C, Yun D-J, Noh EW, Lee H-S, Kwak S-S (2010) Transgenic poplar expressing Arabidopsis NDPK2 enhances growth as well as oxidative stress tolerance. Plant Biotechnol J 9:334347 [135]Shou H, Bordallo P, Wang K (2004b) Expression of the Nicotiana protein kinase (NPK1) enhanced drought tolerance in transgenic maize. J Exp Bot 55: 10131019 [136]Shi H, Ishitani M, Kim C, Zhu JK (2000) The Arabidopsis thaliana salt tolerance gene SOS I encodes a putative Na /H + antiporter. Proc Natl Acad Sci USA 97:68966901 [137]Gaxiola RA, Li J, Unurraga S, Dang LM, Allen GJ, Alper SL, Fink GR (2001) Drought- and salt-tolerant plants result from overexpression of the AVP1 H + -pump. Proc Natl Acad Sci USA 98:1144411449 [138]Zhang HX, Blumwald E (2001) Transgenic salt-tolerant tomato plants accumulate salt in foliage but not in fruit. Nat Biotechnol 19:765768 [139]Shi H, Ishitani M, Kim C, Zhu JK (2000) The Arabidopsis thaliana salt tolerance gene SOS I encodes a putative Na /H + antiporter. Proc Natl Acad Sci USA 97:68966901 [140]Chinnusamy V, Jagendorf A, Zhu JK (2005) Understanding and improving salt tolerance in plants. Crop Sci 45:437448 [141]Century K, Reuber TL, Ratcliffe OJ (2008) Regulating the regulators: the prospects for transcription-factor-based agricultural biotechnology products.Plant Physiol 147:2029
[142]Vincour B, Altman A (2005) Recent advances in engineering plant tolerance to abiotic stress: achievements and limitations. Curr Opin Plant Biol 16:123 132 [143]Lee RC, Feinbaum RL, Ambros V (1993) The C. elegans heterochronic gene lin-4 encodes small RNAs with antisense complementarity to lin-14. Cell 75:843854 [144]Pardo JM, Reddy MP, Yang S (1998) Stress signaling through Ca 2+ /Calmodulin dependent protein phosphatase calcineurin mediates salt adaptation in plants. Proc Natl Acad Sci USA 95:96819683 [145]Mantri NL, Ford R, Coram TE, Pang ECK (2007) Transcriptional profiling of chickpea genes differentially regulated in response to high-salinity, cold, and drought. BMC Genomics 8:303[146]Shinozaki K, Yamaguchi-Shinozaki K (1997) Gene expression and signal transduction in waterstress response. Plant Physiol 115:327334
[147]Liu Q, Kasuga M, Sakuma Y, Abe H, Miura S, YamaguchiShinozaki K, Shinozaki K (1998) Two transcription factors, DREB1 and DREB2, with an EREBP/AP2 DNA binding domain separate two cellular signal transduction pathways in drought- and low temperature-responsive gene expression, respectively, in Arabidopsis. Plant Cell 10:13911406 [148]Ashraf M (2010) Inducing drought tolerance in plants: recent advances. Biotechnol Adv 28:169183 [149]Hsieh TH, Lee JT, Charng YY, Chan MT (2002) Tomato plants ectopically expressing Arabidopsis CBF1 show enhanced resistance to water deficit stress. Plant Physiol 130:618626 [150]Winicov I, Bastola DR (1999) Transgenic overexpression of the transcription factor Alfi n1 enhances expression of the endogenous MsPRP2 gene in alfalfa and improves salinity tolerance of the plants. Plant Physiol 120:473480[151]Sahi S, Singh A, Blumwald E, Grover A (2006) Beyond osmolytes and transporters: novel plant stress tolerance related genes from transcriptional profiling data. Physiol Plant 127:19
[152]Flowers TJ (2004) Improving crop salt tolerance. J Exp Bot 55:307319